The oviduct (Fallopian tube, uterine tube) connects the ovary to the uterus horns and are 20-30 cm long. The oviduct is supported by the mesosalpinx and is very tortuous. Sperm travel up the oviduct prior to fertilization and the embryo travels down the oviduct after fertilization.
http://www.ansci.wisc.edu/jjp1/equine/mare_anat/oviduct1.html
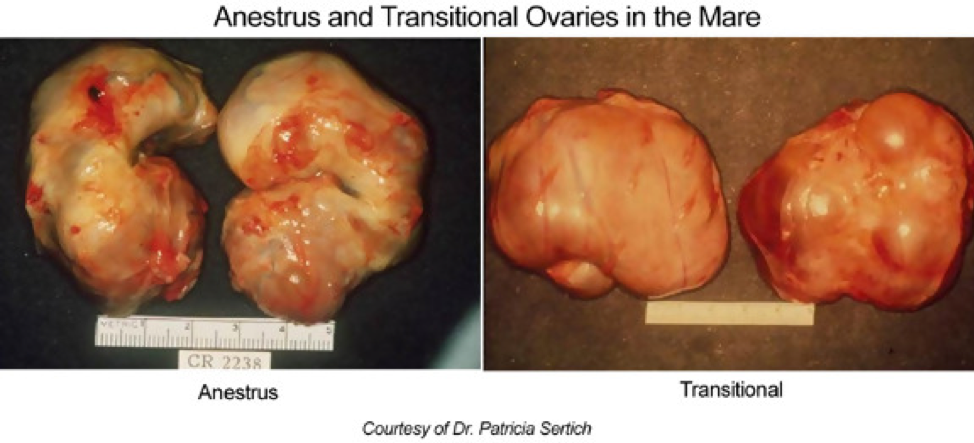
Estrus, or heat, is the period of the reproductive cycle when the mare ovulates and, if bred, is likely to conceive. Estrus is also the time when the mare is receptive and will accept the stallion. The average length of the estrous cycle, or the period from heat period to the next heat period, is 21 days, but the estrous cycle can vary from 19 to 26 days. The duration of estrus is five to seven days (actually about six days), but it can vary from two to 10 days. The first heat following foaling is referred to as foal heat. Foal heat typically occurs six to nine days after foaling, but it may be as early as five days or as late as 15 days.3
https://nexgenvetrx.com/blog/horsebreeding/when-do-mares-ovulate/
Anatomy, Physiology and Reproduction in the Mare
Agdex#: 460/30
Publication Date: 12/10
Order#: 10-099
Last Reviewed: 12/10
History: Replaces the Factsheet of the same name (Order No. 88-060). The original Factsheet was authored by Dr. Bob Wright, Veterinarian-Disease Prevention, Equine and Alternate Species, OMAFRA, retired.
Written by: Updates coordinated by Tania Sendel, Veterinary Science and Policy Unit, OMAFRA
Table of ContentsIntroduction
Estrous Cycle
Seasonality
Hormones
Anatomy
Fertilization
Age
Management
Introduction
Horses have the potential for high reproductive performance. With an understanding of basic reproductive science, breeders can be better positioned to achieve their goals.
This Factsheet presents information on basic anatomy, physiology and management techniques that can assist in improving reproductive performance in the mare.
Estrous Cycle
The estrous cycle in most mares starts to normalize in late April or early May until August - the normal breeding season for horses. During this time, the mare will have an estrous cycle of 21 days (±3 days). The estrous cycle is composed of two phases: the estrous phase (in heat) and the diestrous phase (out of heat). Estrus usually lasts for 6 days, but can be 4-10 days, depending on the mare. Diestrus is normally 15 days, but may vary from 12-18 days. From September through March, very few mares will cycle normally, so conception is more difficult to achieve during these months.
Ovulation, the release of the egg from the ovary, can occur at any time during the estrus phase. However, it normally occurs 24-48 hr before the end of the estrus period. Ideally, to maximize the chance of conception, breeding should occur within 12 hr of ovulation. Breeding or insemination of mares, starting on Day 2 or 3 of estrus and continuing every other day throughout the estrus, is a practical means of achieving satisfactory pregnancy rates.
Seasonality
The mare's first estrus phases of each year are often erratic and prolonged, during which time mares may be in heat for 20-30 days or more. During late March, April and May, most mares exhibit sexual receptivity, and from late April through August, most mares will cycle normally.
Few early estruses result in ovulation; thus it is not recommended to breed mares during this time without rectal palpation of the ovaries to determine follicular development.
The primary environmental factor causing mares to cycle normally is increased hours of light (photoperiod). Artificial lighting can be used to induce mares to cycle earlier in the year.
Hormones
The estrous cycle is controlled by hormones (Figure 1). Hormones are chemical substances created by the body that control various bodily functions. This section describes some of the hormones involved in mare reproduction.
Figure 1. The estrous cycle.
Text Equivalent of Graphic
The pituitary gland is located at the base of the brain and produces follicle-stimulating hormone and luteinizing hormone. As spring approaches, the pituitary gland is stimulated by increased daylight to enhance follicle-stimulating hormone production. Follicle-stimulating hormone is released into the blood stream and travels to the ovaries to initiate development of a follicle containing an ovum. The developing follicle produces estrogens, which are released into the blood stream.
Estrogens have a number of functions in the body. When blood estrogen reaches a certain level, a surge of luteinizing hormone is released from the pituitary gland into the blood stream. Estrogens are responsible for the clinical signs of estrus and act on the oviducts, uterus and cervix to prepare the reproductive tract for pregnancy.
The surge of luteinizing hormone causes the follicle on the ovary to rupture, resulting in ovulation. As the follicle develops on the ovary, the ovum (egg) inside the follicle undergoes a number of changes to become capable of being fertilized by the sperm. The follicle contains a viscous fluid and when the follicular wall ruptures, this fluid flows out, carrying the ovum with it. The cavity left by the ruptured follicle becomes engorged with blood to form a corpus hemorrhagicum. The corpus hemorrhagicum luteinizes to form the corpus luteum, sometimes called the yellow body.
As the corpus luteum develops, it starts to produce progesterone, which influences the pituitary gland and reproductive tract. The feedback of progesterone via the blood stream inhibits the release of luteinizing hormone. Under the influence of progesterone, the mare will not show estrus. Progesterone function is to maintain the pregnancy by maintaining a uterine environment conducive to fetal development.
If the mare does not conceive, the corpus luteum remains functional for about 12-14 days. At this time, prostaglandin is released from the endometrium (inner lining of the uterus). Prostaglandin has a luteolytic effect - it acts on the corpus luteum via the bloodstream, causing it to regress. As the corpus luteum regresses, progesterone levels are reduced, resulting in the removal of the inhibition to luteinizing hormone secretion. The cycle starts over again.
If the mare conceives, hormonal activities are essentially the same as for the 12-14 days post-ovulation. Pregnancy recognition is stimulated by the action of the developing embryo migrating throughout the uterus; this action inhibits prostaglandin release. The result is an antiluteolytic effect, so the corpus luteum remains functional, progesterone levels are maintained and the pregnancy is continued.
Somewhere between Days 25 and 30 of gestation, the corpus luteum starts to regress, resulting in declining blood progesterone levels. If the progesterone level were to continue to decrease, the pregnancy would be terminated. However, a compensatory system has evolved that is unique to the mare. Between the 25th and 36th day of gestation, a girdle-like band of special cells develops around the fetal sac. On about Day 37 of gestation, this band detaches from the fetal membranes and invades the endometrial wall where these cells undergo tremendous enlargement and structural change. These cells clump to form the endometrial cups that secrete the hormone equine chorionic gonadotropin. Equine chorionic gonadotropin reaches the ovaries via the blood stream, stimulating secondary follicular development and luteinization. The secondary corpus luteum produces progesterone, as does the primary corpus luteum to Day 130 to 150 of gestation. From about Day 80 of pregnancy to term, adequate progesterone levels are maintained by special areas of the uterus and fetal membranes, to sustain the pregnancy.
The pregnant mare foals (parturition) at 340 days ±20, post-breeding. Initiation of parturition is very complex and not completely understood, but the fetus probably plays a role in initiating the process. Mechanical stimuli occur from distension of the uterus, which brings about an increased sensitivity of the uterus to the hormones estrogen and oxytocin. At the end of pregnancy, the uterus becomes active and the cervix dilates. Oxytocin, released by the pituitary gland, causes the muscles of the uterus to contract and expel the fetus (foal).
Anatomy
Figures 2 and 3, respectively, depict sagittal and frontal views of the mare reproductive structures.
Figure 2. Sagittal view of the mare reproductive structures.
Text Equivalent of Graphic
Figure 3. Frontal view of the mare reproductive structures.
Text Equivalent of Graphic
Broad ligament - a tough layer of fibrous tissue, containing blood vessels and nerves that serve to suspend the majority of the reproductive tract in the abdomen.
Cervix - a structure of approximately 10 cm (4 in.) in length between the vagina and the uterus. It is the "door to the uterus" and serves to maintain a sterile environment in the uterus. It relaxes when the mare is in heat and closes when not in heat or pregnant.
Infundibulum (fimbria) - the "catcher's mitt" structure at the ovarian end of the oviduct that picks up the ovum from the ovary at ovulation and transports it down into the oviduct.
Ovary - the primary sex organ of the mare. The ovary produces the ovum (egg) to be fertilized and serves as an endocrine gland producing the hormones estrogen and progesterone.
Oviduct - a long, convoluted tube extending from the infundibulum to the end of the uterine horns. It serves to transport sperm and ova to the site of fertilization in the upper one-third of the oviduct. The fertilized ovum is then transported to the uterus.
Uterus - a large uterine body, just anterior to the cervix, and two relatively short uterine horns that terminate in the oviduct. The uterus is where the majority of embryonic development and nourishment takes place. It also produces hormones and is the receptacle where semen is deposited during natural breeding.
Vagina - part of the birth canal that lies in the pelvic girdle between the vulva and the cervix.
Vulva - the external opening of the urogenital tract. It is part of the birth canal, and the area where urine is voided.
Fertilization
The site of semen deposition in the mare is intra uterine (natural breeding) or the uterine body (artificial insemination). The muscular movements of the uterus and oviduct under the influence of estrogens are responsible for the migration of sperm to the oviduct.
When the follicle ruptures, it releases the ovum to be picked up by the fimbria (infundibulum). The fimbria funnels the ovum into the oviduct, where it comes in contact with the sperm. The union of the sperm and ovum forms the zygote, the beginning of an embryo. The embryo moves down the oviduct to the uterus. The time required to move the embryo from the site of fertilization into the uterus is about 6 days. By this time, the uterus has been under the influence of ovarian progesterone to create a suitable environment for fetal development and implantation.
Studies have shown that the embryo is relatively mobile within the uterus until Day 16 or 17, post-ovulation, because of the increased uterine tone, thickening of the uterine wall and enlargement of the vesicle. Movement throughout the uterus plays a role in the inhibition of the mare's estrous cycle.
Implantation occurs around Day 35 of gestation and placentation is initiated around Day 40 to Day 45. Up to this time, the fetal sac lies unattached in the lumen of the uterus.
Age
Horses reach puberty between 12 and 18 months of age. It is therefore advisable to keep colts and fillies separate once they have reached 1 year of age. Even though young horses can reproduce, it is not advisable. Mares that are bred prior to maturity will require extra care and nutrition, especially during the period of lactation and last 3 months of pregnancy (due to the risk of dystocia), so that she and her foal will grow to their genetic potential.
Handle mares and performance test them prior to being bred, to assess their quality. If they perform well, the value of their foals increases.
Management
The mare's body condition will influence her reproductive performance. Mares that are moderately fleshy (slight crease down the back, fat covering the outlines of the ribs, noticeable amounts of fat along the sides of neck and withers, and soft fat deposited around the tail head) can be expected to: cycle earlier in the year
have fewer cycles per conception
have higher pregnancy rates
maintain pregnancy more easily than thin mares
Therefore, preparing a mare for breeding with a feeding program of sufficient, nutritionally balanced, high-quality feed is recommended.
To detect a pregnancy, a transrectal ultrasound is recommended as early as 12-15 days, post-breeding. Also, a veterinarian can conduct a foal-time test between Day 45 and Day 120 to confirm pregnancy. A foal-time test is a serological test that detects the presence of equine chorionic gonadotropin; it does not guarantee that the mare is pregnant at the time of testing, but does indicate whether the mare was pregnant up to Day 37 of gestation. Knowing that a mare is not pregnant allows for planning additional breeding attempts.
Following recommended management practices and understanding the basic reproductive science of the mare will improve chances for reproductive health and success of the mare.
For more information:
Toll Free: 1-877-424-1300
E-mail: ag.info.omafra@ontario.ca
http://omafra.gov.on.ca/english/livestock/horses/facts/10-099.htm
Breeding From Your Mare
Understanding the basic physiology and anatomy of your mare will help you understand what is involved in successfully breeding healthy foals.
Reproductive Anatomy:
Mares have two ovaries. The ovaries are situated in the dorsal (upper) part of the abdomen, just behind the kidneys. The ovaries are the female gonads and they produce and release eggs. They are also the site of production of the female sex hormones oestrogen and progesterone. The uterus (womb) is comprised of two horns and a body, all being suspended from the roof of the abdomen by the broad ligament. The cervix protects the entrance into the uterus from the vagina.
Figure 1: The mare’s reproductive system Figure 2: The mare’s reproductive system in situ


The oestrus cycle:
In the Northern Hemisphere mares are most sexually receptive and active from April through to September. During winter, mares become sexually inactive and are said to be in a state of anoestrus. As spring approaches and day light length increases, the pineal gland within the brain produces less of the hormone melatonin and the mare enters a transitional period. (Sometimes mares can exhibit intermittent grumpy behavior during the transitional period.) With rising hormone levels, follicles within the ovaries are stimulated to grow. Initially lots of follicles grow simultaneously until one becomes dominant and ovulates releasing the egg from the follicle. Following this, regular oestrus cycles will occur throughout the summer months.
Each oestrus cycle lasts around 21 days with the mare being in season (sexually receptive) for 3-8 days. During this time the mare will show variable behavioural changes such as frequent posturing (see below) and she will also be receptive to the stallion, allowing him to nuzzle her.
Figure 3: Mare in season

These behavioural changes occur due to increasing levels of the hormone oestrogen, which is released from the follicles. Ovulation usually occurs 24-48 hours before the mare goes out of season and what remains of the follicle after ovulation forms a structure within the ovary called the corpus luteum (C.L). The C.L. produces another hormone called progesterone. Simply speaking progesterone has the opposite effect to oestrogen and the mare will now be out of season and no longer receptive to the advances of the stallion. At around 16-17 days, if a pregnancy is not detected, the lining of the uterus produces a hormone called prostaglandin, which destroys the C.L. and consequently the levels of circulating progesterone decrease. This reduction in progesterone allows follicles to develop again and then the mare returns to season. However if the mare is pregnant the C.L. will remain and with the help of other hormones, the pregnancy will be supported and there will be no return to osetrous.
Preparing your mare for stud
Prior to sending your mare to stud there are a number of things that need to be considered, such as her having a veterinary examination to ensure that she is fit to breed.
In order to perform this safely your veterinary surgeon may require stocks, although quiet, well-behaved mares can be assessed over a stable door.
A typical breeding soundness examination includes:
• A physical examination
• Rectal examination
• Ultrasound scan
• Reproductive examination
At the same time as the breeding soundness examination the veterinary surgeon will also perform clitoral swabs in order to make sure that the mare is free from the bacteria (Taylorella equigenitalis), which causes the venereal disease Contagious Equine Metritis (CEM). Blood tests are also performed to rule out viral infections, namely Equine Viral Arteritis (EVA) and Equine Infectious Anaemia (EIA) prior to entering the stud.
Pre-stud checklist
Before sending your mare to stud you will need to consider whether the following are required and up to date:
• Vaccinations (tetanus, influenza and herpes virus (many studs stipulate that horses are vaccinated against equine herpes virus in order to minimize the risk of abortion storms)
• Worming
• Dental health
• Routine hoof care
• Overall physical condition of the mare
Most stud farms will impose a 2-week period of isolation for any new arrivals. This allows new arrivals to be monitored for any infections, which they may be incubating, which they may not have been tested for, thus safe guarding the stud farm from potentially serious infections. If your mare is pregnant and you are sending her away to stud to foal, then you should contact the stud for further information, as the mare may well need to arrive at the stud at least 3-4 weeks prior to her foaling date.
Central Equine Vets can provide you with further information on both what is involved with a breeding soundness examination as well as providing advice on appropriate considerations for breeding your mare.
Mating
Mares can be mated or “covered” in one of two ways:
1. Natural mating with a stallion
2. Artificial insemination (A.I.)
With artificial insemination semen is collected from a stallion, which maybe from a different part of the country or a different country all together, thus giving you, the breeder, a wider range of choice of sire. The collected semen is then stored, and at a later date, placed into the uterus of the mare.
Mares are often covered naturally, especially in the thoroughbred industry.
Advances in equine reproductive knowledge and techniques mean that it is now possible for a veterinary surgeon to examine the mare to see what stage of her oestrous cycle she is in. Using modern hormone drugs it is then possible to manipulate the mare’s oestrous cycle in order to induce ovulation and thus mate the mare as close to the ovulation as possible. This means that fewer matings are required.
Artificial Insemination (A.I.)
A.I. involves collecting semen from the stallion and then implanting it into the mare. The semen is collected by having the stallion either mount a teaser mare or dummy. Once mounted, the stallion ejaculates into an artificial vagina (A.V.), and the semen is collected. From here it can be used immediately or it maybe chilled, or frozen, and then transported to the stud farm where the mare is stabled.
The mare is then inseminated once she is in season. The semen is thawed (if frozen) and inserted into the mare’s uterus through the vagina and cervix via an A.I. gun (pipette).
Advantages of A.I.?
• Reduced risk of spreading venereal diseases as there is no direct stallion-mare contact
• Reduced risk of injury to the horses and handlers compared to natural mating
• Achieves mating whilst the sire and dam are on different stud farms, or even in different countries
If you are considering breeding from either a mare or stallion Central Equine Vets will be able to provide you with further advice as to which method is most appropriate for you.
Back to Fact Files
https://www.centralequinevets.co.uk/BreedingYourMare1532.html
Factors Determining Follicle Size in Mares
In mares, follicles typically ovulate at diameters of between 35 and 55 mm. Within the context of a breeding program, it is important to establish baselines for the ongoing measurement of mares' follicles, as well as continuity in determining related measurements. By Day 16 of the mare's cycle, the developing follicle has reached the size at which it secretes enough hormone to bring on the external characteristics of heat.
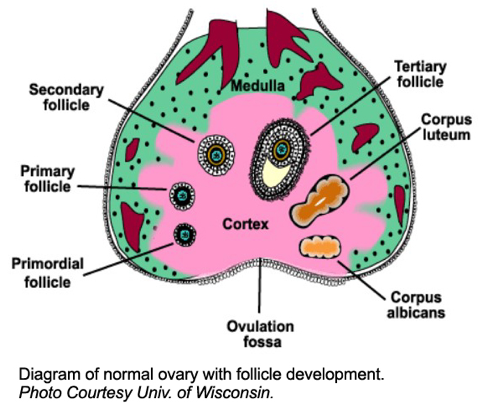
There are several factors that determine the size equine follicles will ultimately attain prior to ovulation. Among these are general health, stage of life, diet, temperament and the presence of existing medical conditions. Then, there is the delicate hormonal interplay which occurs during the estrous cycle.
Typically, preovulatory follicle grow at a rate of 3 mm a day for up to 2 days before ovulation. The size then remains constant, before diminishing by 2-3 mm during the last 12 hours.4
It has been suggested that the follicle destined to be a dominant follicle is the first to reach a critical follicle size of 21-23 mm prior to other follicles and thus creating a condition that inhibit the further growth of the subordinate follicle (Gastal et al., 1997). Several hormones have been demonstrated as potential factors that control the follicle growth and subordination. During a 21-22 day of oestrous cycle, the gonadotropin releasing hormone (GnRH) releases substantially from the hypothalamus and subsequently stimulates the production of the two important gonadotropins (follicle stimulating hormone (FSH) and luteinizing hormone (LH)). FSH plays a key role in the emergence of the ovulatory wave, but the plasma circulating FSH declines when the size of the largest follicle reaches approximately 13 mm. It seems likely that the largest follicle have ability to utilize the low level of FSH, while other factors such as LH, inhibin, insulin-like growth factor 1 (IGF-1) and estradiol also play a role during follicle deviation.1
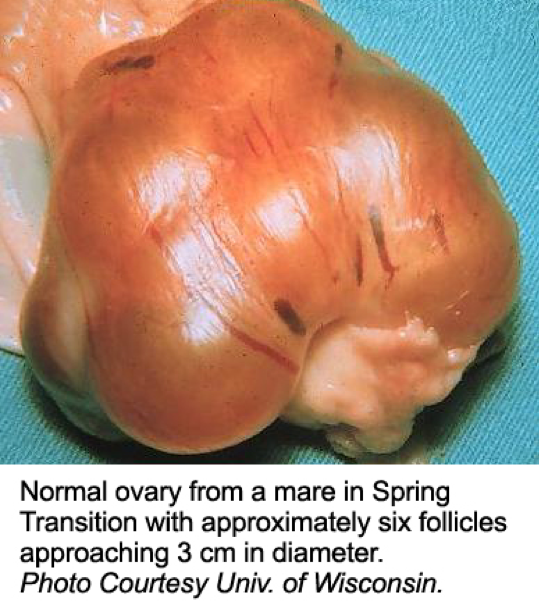
Determination of Follicle Presence and Size
After ovulation, the cells that remain in the follicle divide and begin to produce progesterone. The ovulated follicle forms the corpus luteum (CL). The CL produces progesterone for 14-15 days. If the mare is not pregnant, the CL regresses and stops producing progesterone.3
For mares, rectal palpation by a veterinarian provides critical information regarding the condition of the reproductive tract, and can confirm the presence of a follicle. Further testing, including uterine ultrasound, uterine biopsy, and cultures may be recommended. Finally, the conformation of the vulva should be periodically evaluated to determine if the mare is susceptible to uterine infections, which become more likely as the mare ages.
https://nexgenvetrx.com/blog/equine/breedingproducts/mare-follicle-size-during-ovulation/
Mare’s ovaries appear to be inside-out compared to those of humans. Like a shirt inside-out still keeps you warm, an ovary inside-out still functions. In human ovaries, the outermost part consists of the cortex and the inner part the medulla; in the mare, the medulla surrounds the cortex, while the ovulation fossa is located in the center of the ovary. The ovulation fossa allows the ovary to still ovulate; therefore, the internal structure has little effect on the organism’s ability to reproduce. Fertilization occurs in the oviduct. Humans experience symptoms of pregnancy such as morning sickness shortly after fertilization.
https://heiferinyourtank.typepad.com/theres_a_heifer_in_your_t/2011/08/bigger-isnt-always-better-inside-out-ovaries-in-horses.html
first_page
settings
Open AccessReview
The Mare: A Pertinent Model for Human Assisted Reproductive Technologies?
by
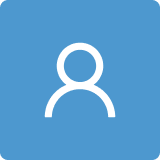
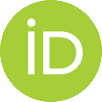
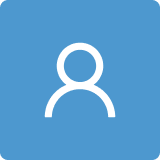
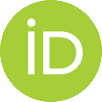
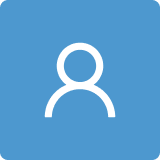
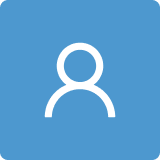
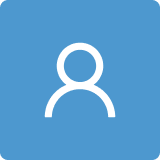
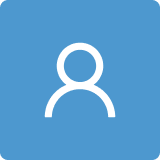
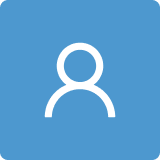
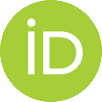
1
Université Paris-Saclay, UVSQ, INRAE, BREED, 78350 Jouy-en-Josas, France
2
Ecole Nationale Vétérinaire d’Alfort, BREED, 94700 Maisons-Alfort, France
3
Department of Gynaecology and Obstetrics, Foch Hospital, 92150 Suresnes, France
4
Académie d’Agriculture de France, 75007 Paris, France
*
Author to whom correspondence should be addressed.
†
Co first authors: these authors contributed equally to the manuscript.
Academic Editor: Katrien Smits
Animals 2021, 11(8), 2304; https://doi.org/10.3390/ani11082304
Received: 27 June 2021 / Revised: 28 July 2021 / Accepted: 1 August 2021 / Published: 4 August 2021
(This article belongs to the Special Issue Challenges in Equine (Assisted) Reproduction)
Download PDF
Browse Figures
Citation Export
Simple Summary
Artificial reproduction techniques (ART) are used widely in human medicine to overcome infertility, with about one in seven couples being concerned in the Western world. Due to ethical concerns, animal models are needed to develop new methodologies. Although laboratory animals are seminal in this context, they have a short lifespan and are usually fertile. Horses are long-lived domestic animals that are bred until old age, often after they have had a career being used for equestrian activities. Their reproductive functions become altered after 20 years, in a similar way to humans, although there is no menopause per se in horses. There is also a concern for rising overweight and obesity concerns in these species. In addition, embryo transfer and ART are developed to overcome infertility, as for humans. This review details similarities and differences in the reproductive cycle, ART, and fertility concerns in women and mares and discusses the opportunity of using the horse as an appropriate model for ART in humans.
Abstract
Although there are large differences between horses and humans for reproductive anatomy, follicular dynamics, mono-ovulation, and embryo development kinetics until the blastocyst stage are similar. In contrast to humans, however, horses are seasonal animals and do not have a menstrual cycle. Moreover, horse implantation takes place 30 days later than in humans. In terms of artificial reproduction techniques (ART), oocytes are generally matured in vitro in horses because ovarian stimulation remains inefficient. This allows the collection of oocytes without hormonal treatments. In humans, in vivo matured oocytes are collected after ovarian stimulation. Subsequently, only intra-cytoplasmic sperm injection (ICSI) is performed in horses to produce embryos, whereas both in vitro fertilization and ICSI are applied in humans. Embryos are transferred only as blastocysts in horses. In contrast, four cells to blastocyst stage embryos are transferred in humans. Embryo and oocyte cryopreservation has been mastered in humans, but not completely in horses. Finally, both species share infertility concerns due to ageing and obesity. Thus, reciprocal knowledge could be gained through the comparative study of ART and infertility treatments both in woman and mare, even though the horse could not be used as a single model for human ART.
Keywords: oocyte; embryo; intracytoplasmic sperm injection; ovum pick-up; maternal age; obesity; exercise; in vitro maturation
1. Introduction
Animal breeding has been performed by humans since prehistoric times. Selective breeding really started in the early 18th century in the UK with Sir Robert Bakewell, who developed objective selection through accurate recording of animal performance and progeny testing [1]. Criteria used to select domestic animals could be classified in different categories: (1) those necessary for human feeding, (2) those necessary for their physical capacities, and (3) those related to aesthetic or behavioral properties. In the first category, product quantity and/or quality, according to species (meat, milk, wool, eggs…), has been the selection criteria. Associated with production and rentability, fertility has also been positively selected because infertile animals were usually culled. With genetic progress being based on reproductive speed, young and fertile animals are used as the selection base. In the second category, the initial objective was to provide labor, which, when automobiles and tractors were introduced, declined in popularity. The third category concerns a specific behavior (specific gait for example) or a particularly praised esthetical trait. In this case, selection will be based on young animals as soon as the phenotype is known, or, like the other categories, as far as the genotype can predict the phenotype of these animals, on the individual’s genotype. Thus, in most selection processes, aged and infertile animals are not considered.
With these selection objectives, in cattle, assisted reproductive technologies (ART) are used commonly to produce embryos, with an increasing number of embryos produced in vitro each year worldwide (>106 in 2019) whereas the number of in vivo derived embryos declines steadily (around 4 × 105 in 2019) [2], with younger animals, even prepubertal, being used to speed up genetic progress in combination with genomic selection. In horses, however, horse meat consumption is not the main focus of horse production. Most horses are bred for sport or leisure, and depending on their use, will reproduce after they have achieved their career, whether as an athlete (i.e., in racing, endurance, show jumping, dressage, steeplechase) or as a leisure horse (i.e., pony and riding clubs, equestrian tourism). Selection for reproduction is based on performance and, depending on the breed, use, and geographical location, genetic indices may be or not calculated and used for selection. Many male horses are gelded at an early age (often 2 years) for management, so that only highly valued animals are kept for breeding. Thus, both males and females may be used for reproduction until they become elderly. In these species, when allowed by the breed’s studbook, ART is used to breed elite infertile and/or older animals, even though genetic progress is hampered when breeding older animals [3].
Regarding humans, it has been estimated that 15% of couples in industrial countries are infertile, with a frequency that continuously increases due to environmental problems and delayed pregnancy [4,5]. Indeed, the chance to conceive per cycle, for women between 20 and 30 years old, has been estimated at 21–28% per cycle and decreases with maternal age. In most of the Western world, women’s age when they have their first child is increasing [4]. To improve human ART, new technologies and research programs are necessary. Due to ethical concerns, there are many limitations to experimentation using human embryos and the evaluation of new technologies is often impossible. Thus, animal models are seminal to progress in human ART. Studying gametogenesis, the mouse model seems the more appropriate, but there are two main limitations: (1) the efficiency of gametogenesis is dramatically different when compared to human and (2) environmental modelling is quite limited in many situations, while in terms of fertilization, the mouse model remains the best model [6]. For the study of embryo development until implantation, the mouse is definitely very different from all other mammals. Appropriate models are still being discussed, with rabbit development being very close to that of human, indicating that these species should be further employed as a model for human ART [7], while the wide use of ART in cattle throughout the world [2] provides insight in short and long term environmental effects of ART [6,8]. The horse has been pointed out as an appropriate model for follicular development and oocyte ageing [9,10], but may be more widely pertinent as a model for humans.
The aim of this review is to evaluate the horse as a model to study human reproduction and particularly to improve ART considering that (1) selection is not based on reproduction capacity [3,11], (2) infertility seems to impact a large number of stallions and elderly mares [12], (3) reduced activity in leisure horses leads to frequent obesity [13,14,15], and (4) pregnancy occurs after sports career retirement at middle age [16].
2. Comparative Anatomical, Physiological and Pathological Aspects of Reproduction in Mares and Women
2.1. Anatomical Considerations: Uterus and Ovaries
The uterus is the maternal organ that receives the embryo. It ensures, through its secretions, its development until implantation. Uterine anatomy differs among mammalian species and is adapted to their reproductive biology, such as trans-uterine migration of blastocysts and litter size. The uterine tissue is composed of an external muscular tunic (myometrium) surrounding an internal glandular layer, the endometrium. The human uterus is simple with one large uterine body, 7 cm long, without uterine horns while the horse uterus is bicornuate with a 5–8 cm straight cervix and a 20 cm long uterine body communicating with two uterine horns about 30 cm long.
Ovarian development occurs during fetal life both in humans and horses, with initiation of meiosis until meiotic prophase taking place in the first half of gestation and folliculogenesis occurring roughly from mid pregnancy until puberty (around 12 years in humans vs. 1 year of age in horses) [17]. In women, the fertility capital, represented by her follicular reserve, is definitively constituted during fetal life and estimated at approximately ± 7 million at 20 weeks gestation [18]. In comparison, a smaller number of primordial follicles (around 36,000 with a high variability among individuals) is observed in 2–4 years old (adult) mares [19].
The woman’s ovary is a smooth organ, measuring about 4 cm long, 2 cm wide and 1 cm thick. The outer stromal connective tissue, called cortex, that encloses follicles, is located below the surface germinal epithelium and the albuginea, whereas the central connective tissue, called medulla, is composed of a hilar zone (containing vessels, nerves…), a parenchymatous zone with loose connective tissue crossed by vessels in relation with the cortex and the rete ovarii (the hilum) (Figure 1). The equine ovary is approximately the size of a chicken egg (around 5 cm in length and 3 cm in width), with a kidney-shape structure. The internal structure consists of a central “ovarian cortex” with follicles surrounded, except for the area of the ovarian fossa, by a very thin tissue corresponding to the “medulla” in other species and humans. The ovarian fossa, in the concave area of the ovary, is the only place where ovulations can occur. The rest of the surface of the gonad is covered by the visceral peritoneum [20].
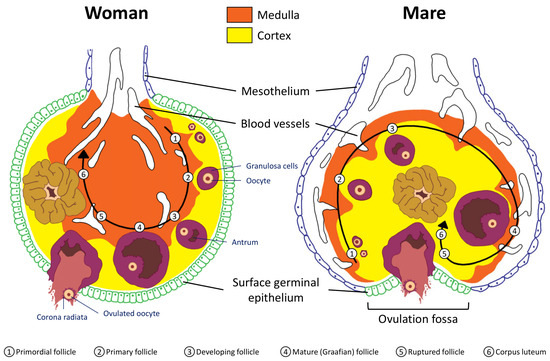
Figure 1. Comparative ovarian anatomy and folliculogenesis in woman and mare (inspired from [20]).
2.2. Folliculogenesis
Folliculogenesis is a long process in which regulatory mechanisms are not well known.
Reproductive and ovarian cycles of women and mares are represented in Figure 2. Conventionally, in women, the cycle lasts 28 days [21], with the first day of the menstrual cycle being the first day of menstruation. Conversely, in mares, the cycle starts on the day of ovulation. An unfertilized mare cycle lasts on average 22 days, including 5–7 days of estrus at the end which ovulation takes place. Without fertilization, luteolysis begins after 12 days post ovulation [20]. In addition, the mare is a seasonal mammal with resumption of cyclicity associated with increased day length [22,23].
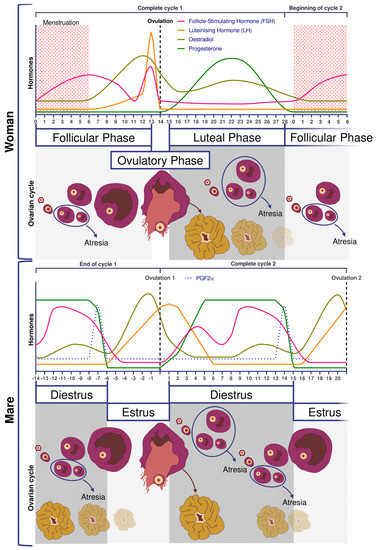
Figure 2. Comparative ovarian and hormonal cycles in women and mares.
In women and mares, at puberty, follicular growth resumes due to the maturation of the hypothalamic-pituitary axis which leads to the secretion of Follicle Stimulating Hormone (FSH), and Luteinizing Hormone (LH) and the stimulation of follicles ≥2 mm in a cyclic manner (reviewed in [21] for humans and [24] for horses). Regularly, a group of primordial follicles starts growing to reach the pre-antral stage (0.1–0.2 mm). As also described in horses, this first phase of follicular growth is independent of gonadotropin support [25]. Once a 0.2–0.4 mm diameter is reached, the antrum begins to develop and follicles become responsive to gonadotropins [25,26]. Follicular depletion begins from fetal life and continues during childhood and throughout reproductive female life in both species [27]. The entire duration of human folliculogenesis is still unknown in both species but has been estimated, from the primordial phase to the preovulatory phase, to span over 200 days in humans [28].
Follicular dynamics are remarkably comparable between humans and horses, with the final dominance of one follicle [26,29]. In both species, follicular growth is due to more complex mechanisms through successive follicular waves (2–3 in horses) that may or may not lead to the development of a dominant follicle that will become ovulatory or not [29,30]. These waves can be described as minor (without appearance of a dominant follicle), major (appearance of dominant follicle ≥10 mm diameter), or as alternating between one and the other in a way that seems random [29,31]. A single dominant follicle is selected in the middle of the major follicular phase and deviates from the growth trajectory of the other follicles until it ovulates, while all other subordinate follicles regress (also known as follicular deviation) [32].
In women, FSH concentrations are low during the luteal phase and rise at the beginning of the follicular phase. On day 7, FSH level begins to decrease (i.e., end of the FSH window), leading to the dominance of only one follicle, generally the biggest one, whose growth is FSH independent [18]. In mares, pronounced FSH surges are observed in the middle of the diestrus.
The diameter of the largest follicle at time of deviation and the maximum diameter of the preovulatory follicle is consistently 2.1 times greater in the mare compared to the woman [31] (around 2 cm in women and >4 cm in mares).
2.3. Ovulation
Ovulation is preceded by an increase in plasma concentrations of estradiol, FSH, and LH, beginning slightly before follicular deviation in both mare and woman (Figure 2) [31]. When the dominant ovarian follicle produces enough estradiol (plasma concentrations of total estradiol in the range of 200–300 pg/mL [33] and about 10 times less in horses [34]), this induces a negative retrocontrol on the hypothalamus conducive to a reduction in FSH secretion. The dominant follicle becomes autonomous and the others undergo apoptosis. Hypothalamic kisspeptin and GnRH secretion increase, resulting in a surge in pituitary secretion of LH roughly at the time of peak estradiol concentrations.
Characteristics of the horse include estrous behavior, characterized by sexual attractiveness to stallions and mating behavior in mares. The drop in plasma progesterone concentrations is a prerequisite for the rising estrogen to induce estrus. Estrus can last up to 6–7 days, with ovulation occurring around 24 h before the end of estrus. Among other differences between the two species, there is no FSH peak at the time of ovulation [35], whereas in women the LH surge is associated with small increases in FSH, with a similar time course [31].
In both mares and women, there is usually one ovulation in normal conditions but double ovulations are frequent. Double ovulations are more of a concern in horses as twin pregnancies are considered as pathological as most result in abortion. They occur in about 20% of the cycles in mares, but this differs according to breed [36] and increases significantly with mare’s [37,38] and women’s age [39].
If fertilization does not occur, luteolysis takes place, thus starting a new cycle. In women, luteolysis is not dependent on uterine prostaglandin secretion as hysterectomized women have normal hormonal curves [40]. However, the lack of hCG and so far still undescribed mechanisms will induce luteolysis [41]. In the horse, as for other domestic animal, luteolysis is dependent on the uterine production of prostaglandin a (as reviewed by [42]).
Anovulation is one of the main causes of infertility in women and females of many domestic species. One of the causes of anovulation, common to both the women [43,44] and mares [45,46,47], is luteinized unruptured follicle (LUF), the precise pathophysiology of which has yet to be determined. LUFs are highly repeatable across cycles (79–90%), resulting in recurrent anovulation [48,49] and infertility in humans. This ovulatory dysfunction has been documented to occur in 11–23% of women with normal menstrual cycles [50,51], 13–73% of women with endometriosis [52], and 4–58% of women with unexplained infertility [53,54]. In cycling mares, LUFs, also known as HAFs (Hemorrhagic Anovulatory Follicles), are also present in 5–20% of estrous cycles [46,47,55,56]. A 5% incidence of LUFs has been reported during the early ovulatory season, followed by 20% during the late reproductive season [46,57], and it seems to occur more frequently in older mares.
In primates, including humans, the ovulated eggs adhere with their cumulus mass of follicular cells to the surface of the ovary. The fimbrial end of the tube sweeps across the ovary to retrieve the egg. Entry into the tube is facilitated by muscular movements that bring the fimbriae into contact with the surface of the ovary. Although there is a small negative pressure in the tube in association with muscle contractions, this does not condition the recovery of the oocytes by the tube [58]. In horses, the uterine extremity of the ovary is attached to the uterus near the tip of the uterine horn by the utero-ovarian ligament, which forms the ovarian bursa that faces the ovulation fossa (Figure 1). This structure considerably reduces the risks of ectopic pregnancy, which is extremely rare [59].
2.4. Preimplantation Embryos
In humans, the acellular zona pellucida surrounds the ovum at ovulation and remains in place until embryo hatching prior to implantation. In horses, an outer gelatinous layer surrounds the oocyte. It is assumed that it consists of cytoplasmic debris from granulosa cells [60] and it disappears after fertilization so that only the zona pellucida still protects the equine embryo at this time [61,62].
In vivo, fertilization takes place in the oviduct. The timing of the different stages of embryo development is depicted in Figure 3 and is very similar between the two species. Fertilized oocytes undergo their first cleavage during the first 24–27 h post fertilization. Next cell divisions occur quickly: the embryo reaches the 4–6 cell stage within 44–48 h and the 16 cell stage within 68–72 h after fertilization [61,63].
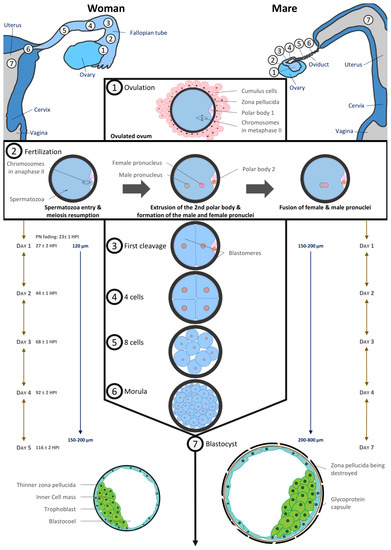
Figure 3. Comparative embryo development in Human and equine (inspired from [20,87]).
The culture of human embryos in time-lapse imaging systems has allowed more recently to estimate more precisely the timing of cell divisions linked to blastocyst formation and embryo potential in terms of implantation [64]. During early cleavages, the human embryo needs more lactate and pyruvate as energetic substrates to develop [65].
Translation of maternal transcripts within the human embryo begins very early: DNA synthesis activity can be detected 9–10 h after insemination [66]. Embryonic genome transcription begins between the 4- and 8-cell cleavage stages, i.e., 2–3 days after fertilization [67]. Earlier embryonic signals can be detected shortly after fertilization. As for humans, at the 4th cell stage (6–8 cell stage), the nucleolus of equine embryos is reorganized and embryonic transcription begins [68].
The size of the equine conceptus does not increase during the early stages of development, remaining on average less than 200 µm [61,69]. At this developmental stage, equine embryo carbohydrate metabolism requires as much pyruvate as glucose [66]. By day 7 post ovulation, the blastocoel is formed, and equine embryos have become blastocysts: blastomeres having differentiated in the inner cell mass regrouped in a compact round zone and the trophectoderm, an epithelium that surrounds the entire embryo [61,62,67]. By day 8, the equine blastocyst cavity is entirely layered by endodermal cells [70].
Embryo development is quite similar in humans with a compaction of embryonic cells on day 4 when the embryo reaches around 16 cells. Blastulation starts on day 5 with the individualization of two distinct cell lines which are trophectoderm cells lining the blastocoelic cavity and inner mass cells. Until the morula stage, cell divisions are said to be reductive because they take place at constant embryonic volume within the zona pellucida (around 120 µm). From day 4, embryo metabolism switches towards the consumption of glucose and amino acids [71,72].
A complex transport system ensures the transport of the fertilized human oocyte to its implantation site, the uterus, by means of three different components, (1) ciliary movement, (2) muscle contractility, and (3) tubal fluid, all of which contribute in varying degrees to efficient tubal transport. Various hormonal and neural factors have been shown to modulate ciliary activity, including adrenergic and cholinergic stimulation, ovarian steroids, prostaglandins, angiotensin II and adrenomedullin. It is difficult to access precise timing data in humans, but it has been described that the first embryonic cleavages occur during transport through the tube. The embryo migrates from the location of the fertilization in ampulla to the isthmus and reaches the uterine cavity at blastocyst stage on day 5. During its migration to the uterus, the zona pellucida prevents the embryo from prematurely adhering to the oviduct rather than traveling to the uterus [73].
The mare is unique in that only fertilized oocytes (developing embryos) enter the uterus, approximately 5.5–6.5 days following ovulation [74,75,76], i.e., at the late morula/early blastocyst stage [61,66,76]. Indeed, prostaglandins E2 production by equine embryos in the oviduct induces oviductal muscle relaxation in the isthmus [77,78,79,80]. As unfertilized oocytes do not produce prostaglandin E2, they are retained in the oviduct for several estrous cycles. These unfertilized oocytes are at the origin of masses in oviducts [81].
In most species, time spent in the oviduct is a prerequisite for full development. This may not be the case in humans as successful pregnancies have occurred in humans following the Estes procedure, in which the ovary is transposed into the uterine cavity in case of tubal infertility [82,83]. Moreover, embryo transfers in the uterine cavity of patients are frequently performed at cleavage stages and some ART centers reported transfers at zygote stage with relatively good chances of pregnancy [84,85]. In equine, embryos have been shown to survive in the uterus as early as four days after fertilization [67].
The blastocyst stage is the last common developmental stage between horses and humans. The human blastocyst implants around 7–10 days post fertilization. The zona pellucida becomes thinner as the blastocyst expands and finally ruptures to allow blastocyst hatching before implantation while the blastocyst is still a hatched blastocyst and the uterus receptive. Conversely, in horses, the blastocyst remains free and continues to grow. Under the influence of uterine contractions, it moves in the mare’s uterus until 16–17 days post ovulation, when uterine edema is such that movement stops. It has been shown that the movement of the embryo is involved in maternal recognition of pregnancy, although the exact signal remains controversial (for review [86]). Implantation begins at 35–40 days after ovulation (for review [87]), and therefore an important part of embryo organogenesis begins before its implantation, unlike in human [20].
2.5. Menopause
Menopause is a human term, literally corresponding to the fact that menstruation stops when women get older. Therefore, this term is not literally applicable to other species, such as for horses. It corresponds, however, to ovarian dysregulation and final reduction of the oocyte pool, with a decrease starting during fetal period, and gradually after puberty. Such a biological process has also been described in horses [88,89]. Indeed, it has been estimated that mares reach ovarian senescence on average at 25 years old [20]. Moreover, around 17% of mares older than 20 do not ovulate any more [88,89]. In non-human mammalian species that have been studied, only mares seem to be affected by this process, partly because of the prolonged lifespan in this species.
3. Comparison of Assisted Reproduction Techniques in Horses and Humans
3.1. Ovulation Stimulation
The stimulation of ovulation is routinely used in humans whereas it remains a poorly successful procedure in horses. One of the advantages of stimulation is that mature oocytes are obtained. The retrieval of immature oocytes from unstimulated follicles is widely used in equine and is also possible in humans, but live birth rates after in vitro maturation remain much lower in humans (see Section 3.3).
In humans, the first birth after IVF, Louise Brown, was reported in the UK in 1978 by R. Edwards and Pr. P. Steptoe [90]. In the early 1980s, births after IVF cycles, unstimulated or stimulated with clomiphene citrate, were reported in different countries. Given the low pregnancy rates, the use of urinary gonadotropins emerged in the USA [91] but results stayed not satisfactory. For this reason, the downregulation of endogenous gonadotropin synthesis resulting from the co-administration of gonadotropin-releasing hormone (GnRH) agonists was introduced in the late 1980s and rapidly became the standard of care. Timely induction of final oocyte maturation during the late follicular phase and prior to oocyte retrieval was induced by a single bolus dose of human chorionic gonadotropin (hCG). Commercial gonadotropins have diversified (urinary or recombinant) and stimulation protocols have evolved considerably with the use of GnRH agonists or antagonists and a trigger by antagonist or hCG. Subsequently, randomized controlled trials involving co-treatment with GnRH agonists and antagonists found similar IVF success rates, with a lower overall gonadotropin consumption and reduced rates of ovarian hyperstimulation (OHSS) [92,93]. In contrast, in horses, many treatments, including equine pituitary extracts (EPE), equine chorionic gonadotropin (eCG), gonadotropin releasing hormone (GnRH), as well as immunization against inhibin and partially purified equine FSH (eFSH) have been used with little success to try and superovulate mares [94]. More recently, recombinant equine FSH and LH have been demonstrated as able to increase ovulation rates and embryo recovery in mares, but defined, repeatable protocols still need to be developed [95]. Thus, in vitro oocyte maturation (IVM) becomes compulsory to increase in vitro embryo production from in vivo collected oocytes, as only one or two preovulatory follicles per cycle are available for puncture (see Section 3.2).
3.2. Oocyte Collection and Ovum Pick up (OPU)
To produce embryos in vitro, the first necessary step is oocyte collection. In women, collection from live donors is the only ethical way to access oocytes, whether from the infertile patient or an oocyte donor. This procedure is performed by transvaginal follicular puncture under ultrasonographic control in both species. In horses, oocytes can be collected from live or dead animals (i.e., most commonly, collection of ovaries at the slaughterhouse). Oocyte collection from a known donor is essential to control the full genetics of the in vitro produced embryos in animals. Like in women, OPU is performed for live animals. Moreover, in horses, many laboratories offer the service of oocyte collection and ICSI after the shipment of the ovaries of valuable mares that have died unexpectedly.
Transvaginal ultrasound-guided follicular aspiration was first described in humans in 1983 [96,97], shortly before the first transvaginal OPU was successfully achieved in horses [98]. This procedure is carried out routinely in human IVF [96,97] and veterinary laboratories for its simplicity and effectiveness. In humans, OPU follows ovarian stimulation and is scheduled between 34 and 37 h after the administration of hCG. It is performed as an outpatient procedure under conscious sedation with local epidural, spinal, or general anesthesia in women. In horses, follicular growth is monitored by ultrasound during the cycle, taking advantage of the follicular waves described above, to reach the optimal number of follicles >1 cm diameter to be punctured [99,100]. This allows for the recovery of 5–12 immature oocytes per OPU cases, whereas <1 is obtained when targeting only preovulatory follicles [100,101]. An important detail is that the oocyte in equine is deeply embedded in the follicular wall [102], making it necessary to repeatedly scrape off the follicular wall in order to retrieve the oocyte and lengthening the collection process [103].
The OPU procedure is generally very well tolerated in both women and mares [97,99,104,105,106]. In horses, it can be repeated every two weeks in routine management [106].
3.3. Oocyte In Vitro Maturation (IVM)
Mature, oocytes (metaphase II) together with matured cytoplasm are needed to achieve successful fertilization. In many mammalian species, including horse and human, oocytes collected from immature follicles cultured in vitro can progress to the metaphase II stage, using a procedure known as in vitro maturation (IVM), that takes from 24 to 38 h, depending on species.
Ironically, although the first human embryo generated in vitro was obtained from immature oocytes matured in vitro [107], this technique is still considered as experimental and is not the currently standard ART procedure. The use of this approach in the early days of reproductive medicine (first human live birth after IVM [108]; first live birth from a woman after IVM with her own oocytes in 1994 [109]) was justified to circumvent non-controlled stimulation protocols, timing of ovulation, and the difficulty of harvesting mature oocytes from large pre-ovulatory follicles. With the development of controlled ovarian stimulation (COS) protocols, the use of in vivo matured oocytes for fertilization became the gold standard. Because ovarian stimulation is not efficient in the horse, IVM is used routinely. Factors affecting oocyte maturation in vitro and in vivo in horses have been reviewed extensively [14,110]. In the horse, maturation media differ between laboratories [99,111]. It is important to note that, in horses, oocytes that have just started cumulus expansion are the most able to mature in vitro to metaphase II compared to oocytes with compact cumuli [103].
In humans, IVM indications have now been extended to patients with contraindication to ovarian stimulation, such as severe overstimulation syndrome in case of polycystic ovary syndrome (PCOS) [112,113] or in the case of hormone-dependent cancers before preserving fertility [114]. This alternative is also offered to some patients with poor ovarian reserve or in whom puncture performance after conventional IVF was low, even if the benefit of this strategy remains controversial [115,116,117]. More recently, IVM has been reported as the only therapeutic alternative for patients with FSH resistance syndrome for whom conventional IVF is totally ineffective [118]. It would seem that, depending on the genotype and/or phenotype of these patients, IVM could have a real place in the management of their infertility [119]. Nevertheless, even though IVM techniques have been improved, the lack of data comparing live births [120] or miscarriage rate [121] after IVM or standard IVF explain why the use of this technique remains relatively anecdotal (0.0003% in European ART centers) [122]. In horses, PCOS has not been reported and the horse could thus not be a spontaneous model for this disease.
3.4. Oocyte Manipulation
As explained above, mature oocytes are most frequently used in humans whereas immature oocytes are collected in horses to subsequently undergo IVM before fertilization.
In horses, transport of immature oocytes is performed preferably at 15–18 °C in buffered holding media using special packaging [99,123,124].
Both human and equine oocytes collected from dominant preovulatory follicles need to be kept at 37 °C to avoid damage [125]. It has been suggested that human microtubule spindles are thermosensitive [126] and that changes in temperature can irreversibly affect spindle integrity [127]. The meiotic spindle plays a major role in the successful completion of meiosis by controlling chromosomal movements throughout the stages of meiosis. Disturbances of meiotic spindles have been suggested as influencing chromosomal segregation and subsequently oocyte aneuploidy. Previous studies have shown in humans that the absence of the meiotic spindle is associated with poor fertilization rates and low embryo development [128]. Temperature fluctuations, which can easily occur in routine ART as well as in veterinary practice, may result in major abnormalities of chromosomal distribution.
Furthermore, human oocytes appear to have very limited ability to combat alkalosis. Therefore, pH (pHi) regulation or regulatory capacity may be impacted by in vitro culture conditions although there may be slight species variations in oocyte intracellular pH (reviewed by [129]). pH is known to affect elements of the actin cytoskeleton of the embryo and the oocyte cytoskeleton is responsible for meiotic spindle positioning. Interestingly, the surrounding cumulus cells transmit the ability to regulate pHi to the enclosed oocyte via gap junctions. As a result, denuded mature metaphase II oocytes are incapable of actively regulating pHi [129]. Therefore, in some clinical IVF procedures, such as intracytoplasmic sperm injection (ICSI) or pre-freezing oocyte denudation, in which cumulus cells are removed, the oocytes are extremely sensitive to pH disturbance up to several hours after fertilization.
The gamete intrafallopian transfer (GIFT) technique (transfer of both oocyte and sperm in the oviduct) was used for a long time to treat idiopathic infertility in women. The improvement of in vitro fertilization techniques with embryo transfer and intrauterine insemination, both with increased pregnancy rates and reduced risk of twin pregnancies, however, has made this technique obsolete and almost non-existent nowadays [130]. In horses, oocyte transfers or GIFT in oviducts of synchronized recipients were used as an alternative to embryo transfer for mares (and stallion) that had history of reproductive failures, i.e., failed to produce an embryo or pregnancy, with ovulatory problems, persistent uterine infection, or pyometra (reviewed in [121]). As in humans, however, with the progress made in ICSI, oocyte transfer or GIFT are no longer used [131,132,133], although this technique could still be of interest for research.
3.5. In Vitro Fertilization (IVF) and Intracytoplasmic Sperm Injection (ICSI)
In most species, including humans, in vitro fertilization (IVF) is the most standard procedure to produce in vitro embryos.
Since its introduction in ART in the 1990s [134], ICSI has been rapidly incorporated into routine human IVF laboratory practices. According to the European registry published in 2016 by ESHRE, the share of ICSI in the 2015 activity in the different European countries was 76.7%. Some countries, such as the Czech Republic and Moldova, now only perform this technique [122]. Some intractable indications for ICSI were clearly established from the beginning as the technique itself was developed to overcome the inefficiency of conventional IVF for severe male infertilities, such as severely impaired sperm characteristics, including severe oligospermia, necro or cryptozoospermia, severe asthenospermia or akinetospermia, or some severe teratozoospermia like globozoospermia. In some cases, it is used as a second resort, when there has been a history of fertilization failure in conventional IVF with normal sperm parameters. In addition, this method of fertilization has made possible the use of surgical sperm, particularly testicular spermatozoa, in cases of azoospermia, which affects 1% of men and 10–15% of infertile men [135]. Subsequently, the indications for ICSI in men have been extended to other couples not concerned with male infertility, but for whom ICSI fertilization has become compulsory because it is the only method compatible with the practices or techniques otherwise used in the management of couples, such as: (i) IVM and IVF after warming frozen oocytes (as in the case of oocyte donation) where the resulting denudation of the oocytes makes conventional IVF unfeasible; (ii) in case of preimplantation genetic testing (PGT) which could be performed in association with ART to analyze the DNA from embryos (cleavage stage or blastocyst) to determine genetic abnormalities [136], where ICSI is preferred to conventional IVF as it avoids sample contamination from cumulus cells, extraneous sperm attached to the zona pellucida, and non-decondensed sperm within blastomeres that can affect the accuracy of genetic analysis [137]; (iii) PGT performed after biopsy and embryonic cell sampling and for which the presence of spermatozoa attached to the oocyte results in contamination of the sample with paternal DNA. Finally, the ICSI technique may sometimes be the only one used in certain ART centers or certain countries for purely economic reasons, where the treatment of couples is expensive and where it is considered, rightly or wrongly, that it is not reasonable to take the risk of fertilization failure in IVF such as Middle Eastern countries [138]. However, some studies show that there is no benefit in performing ICSI compared to IVF in the absence of male factors [139] and even live birth rates are lower with ICSI than with IVF by 10% [140]. Consequently, some learned societies have issued recommendations to avoid excessive recourse to ICSI in the absence of male infertility factors [141].
In horses, the first and only two foals born after IVF were produced using a mature oocyte collected from a preovulatory follicle [98] followed by surgical transfer of the embryo in the oviduct, 24–60 h after fertilization [142]. Because equine sperm does not easily penetrate the zona pellucida of in vitro matured oocytes due to zona pellucida hardening and because the partial or total withdrawal of the zona pellucida results in polyspermy [143], ICSI is the preferred ART procedure in equine, when in vitro production is needed due to male or female infertility, or in the case of extremely limited sperm numbers. This procedure is performed by a rising but still limited number of laboratories throughout the world, thus requiring oocytes and frozen semen to be shipped to these structures. Detailed procedures have been described, with better outcomes being obtained using PiezoDrill [99,111,144].
3.6. Embryo Development and Transfer
In humans, embryo transfer is a response to a parental project and the embryo can be transferred in the days following the OPU (after OS or IVM) or after cryopreservation. Depending on the country, the embryo can be solely transferred in its genetic mother or, in some cases, in another woman, whether through embryo donation or as a surrogate mother. In contrast, embryo transfer in domestic mammals is a way to exchange the genetics of animals without moving live animals. It reduces costs and enables sanitary control. In the equine industry, the equine embryo business concerns both OPU-ICSI produced or in vivo recovered embryos throughout the world.
In humans, since the early days of in vitro embryo culture, many modifications have been made to culture systems to optimize the culture environment and increase the yield and number of embryos of good potential available for embryo transfer (ET). This has resulted in most laboratories now culturing embryos under conditions that allow a culture until the blastocyst stage and to obtain high potential embryos with improved overall pregnancy rates. At the same time, the methods for selecting embryos eligible for in utero transfer have become more efficient, which has made it possible to promote single embryo transfers and reduce the time to pregnancy and live birth.
Unlike humans, the equine embryo is often transferred in another mare than the genetic mother. In vivo embryo collections are thus commonly performed in equine. In the 1970s, in equine, firstly surgical and then nonsurgical embryo recovery procedures were developed [145,146]. Initially, embryo transfers were reserved for old infertile mares, but the technology was rapidly extended to mares in competition, mares who foaled late (so as to produce the next foals earlier in the following year) and pubertal two-year-old mares. In vivo, the horse embryo is collected in the uterus at the blastocyst stage (day 6–8 post ovulation). Both in vivo produced and in vitro produced equine embryos are transferred at the blastocyst stage.
Culture conditions are a major concern for embryologists. Indeed, even if the gametic potential has a significant impact on embryonic development, it is accepted that suboptimal culture conditions can be deleterious and decrease the chances of pregnancy by impacting the number of available embryos and the intrinsic developmental potential of the embryos up to the adult age [147]. In humans, an international consensus has recently begun to emerge [148,149]. Air quality in manipulating rooms is a major concern. The laboratory buildings must be designed to ensure an effective overpressure and air renewal while limiting polluting agents such as microorganisms but also volatile organic compounds [148]. Culture conditions (pH, CO2 and O2 pressure, temperature, culture media, hygrometry, and asepsia) are critical and can impact embryonic potential and modify epigenetic marks in the preimplantation embryo [150,151]. In horses, so far, there is no international consensus on embryo culture conditions and several methods have been used successfully since the 2000s (reviewed in [152]). In any case, one way to control the culture environment in both human and equine is to monitor key performance indicators, such as fertilization rate, cleavage rate, blastulation rate, and good potential embryos rate, for which thresholds and benchmark values have been described [153].
It is relatively easy to obtain a good development of embryos until the blastocyst stage in human, whereas stage 8–16 cells can be reached in the horse with a variety of in vitro conditions, but blastocyst formation is more difficult to obtain. Equine in vitro development is delayed compared to in vivo produced embryos: the 8–16 cells stage is only reached at four days post fertilization and the blastocyst stage between seven and 10 days post fertilization (see [154] for review). In addition, expanded blastocysts with a thin trophoblast layer and a distinct inner cell mass, such as in vivo produced blastocysts, cannot be obtained [154]. The zona pellucida does not get thinner and a full capsule surrounding the embryo is not produced in vitro [152,155]. After the transfer of in vitro produced equine embryos into recipient mares, however, the inner cell mass becomes apparent and a complete capsule is formed [156], demonstrating that embryo culture conditions are still suboptimal for equine development and more studies are required.
Another challenge both in human and horses is to select the embryo to be transferred fresh or to be frozen to reduce the time to achieve pregnancy while limiting the risk of discarding viable embryos from the attempt. In humans, in recent years, many tools have been developed to assist medical teams in this selection, while in horses, the quality assessment of cultured embryos is at its beginning. Methods can be invasive, such as embryo biopsy for pre-implantation genetic testing [124,157,158], or non-invasive, such as embryo monitoring by time-lapse imaging [159,160,161,162,163], metabolomics, or aneuploidy diagnosis on spent culture media (niPGT–A) [164]. These different techniques present different sensitivities, specificities, and acceptability, but have allowed to better control early embryonic development, to refine the criteria for the choice of the embryo to be transferred, and to promote single embryo transfer and thus considerably reduce the risks of multiple pregnancies (see below).
3.7. Embryo Biopsy
Genetic diagnosis of human embryos was initially developed and designed to avoid the transmission of serious X-linked diseases (as adrenoleukodystrophy, X-linked mental retardation and Duchenne muscular dystrophy). Embryo biopsies at the early cleavage stage were first performed from 1989, taking one or even blastomeres for preimplantation genetic testing (PGT) of all embryos on day 3. The first live birth following this manipulation was obtained in 1990 [165]. Nowadays, PGT might be reliably adopted to test human embryos from IVF cycles for monogenic diseases (PGT–M), chromosomal structural rearrangements (PGT–SR) and aneuploidies (PGT–A; previously also known as preimplantation genetic screening, PGS). The development of extended embryo culture until blastocyst stage has enabled to perform biopsies on day 5 or 6. Several trophectoderm cells are biopsied, thus leaving the ICM intact and significantly increasing the number of cells and consequently DNA quantity obtained for genetic analysis. The biopsy of the first polar body (PB) was also proposed to diagnose maternally transmitted monogenic diseases, later complemented by analysis of the 2nd PB obtained after fertilization [166]. This technique is less invasive and allows an additional two days for genetic analysis before reaching the day 3 stage and possibly performing a fresh embryo transfer. In the event of an inconclusive result, it is still possible to perform an embryo biopsy at day 3 or at the blastocyst stage.
In horses, embryo biopsy is performed in morulas and blastocysts, passing through the zone pellucida and collecting cells in the periphery of the embryo to avoid the inner cell mass that is not visible in morulas. For older embryos, a PiezoDrill is used to breach through the capsule and trophoblast cells are collected, far away from the inner cell mass [167]. This technique is widely used commercially for embryo sexing [124,168], as well as in genetic evaluation for embryo selection [111,169,170].
Nowadays, the improvement in the efficiency of high-throughput sequencing techniques with the next generation sequencing (NGS) in both species and, in the human, the improvement in blastocyst freezing/warming methods with the advent of vitrification has clearly contributed to the generalization of embryo biopsy both in humans and horses and the extension of their indications: improved pregnancy rates, reduction of the transmission of serious diseases in humans [171], but also sex determination and genetic evaluation in horses. The next challenges rely on the development and use of less technically challenging and/or non-invasive techniques to perform genetic testing both in humans and horses [111,172,173].
3.8. Oocyte and Embryo Cryopreservation
Cryopreservation programs are crucial to optimize ART protocols, safety, and efficiency. The greatest challenge during cryopreservation of embryos and oocytes is to prevent ice crystal formation and avoid cryoprotectant toxicity, main causes of cell death associated with cryobiology, while maintaining the cellular functions and viability of the embryo or oocyte [174]. In humans, embryo cryopreservation has decreased the number of fresh embryos transferred, and thus the occurrence of multiple pregnancy. Oocyte freezing has revolutionized fertility preservation, whereas in horses, embryo cryopreservation is commonly used for embryos produced in vitro but is still not efficient for in vivo produced embryos.
The first pregnancy and birth after transfer of frozen-thawed human embryos were reported in Australia in 1983 and 1984 [175,176], at the same time as the first successful cryopreservations resulting in live foals [177]. In both species, various protocols have been proposed using different types and concentrations of cryoprotectants, equilibration timing, cooling rates, and cryopreservation devices (reviewed in [123,178,179]). For almost 20 years, slow freezing was the only technique used in humans. In contrast, in horses, slow cooling was not widespread due to the poor success and the financial investment needed. Major factors affecting the success of cryopreservation of equine embryos are indeed their size, their large blastocoel and the glycoprotein capsule that reduces the penetration of cryoprotectants. Embryos smaller than 300 µm freeze well (pregnancy rates around 50–60%), while larger embryos result in poor pregnancy rates (around 20–30%), whether with slow cooling or vitrification (for review [179]). Thus, so far, most embryos are transferred fresh. Nevertheless, the recipient mare may be far away from the laboratory, so embryos are shipped all over the world in specially designed cooling containers. Successful procedures for equine embryo cooling were developed in 1987 [180] and are still effective, enabling successful transfer within 24 h of collection.
Vitrification, that allows the solidification of cells into a glass-like state without the formation of ice, has supplanted slow freezing in humans over the last ten years and is increasingly used in horses. In humans, available data suggest that vitrification/warming is superior to slow freezing/thawing with respect to clinical outcomes and cryosurvival rates either for oocytes, cleavage-stage embryos and blastocysts (reviewed in [181]). The high results of vitrification, particularly at blastocyst stage, have made it possible to change clinical practices with differed embryo transfer (freeze-all strategy) to avoid ovarian hyperstimulation syndrome with identical to superior results [182]. In equine, vitrification has proven very successful for in vitro produced embryos that are smaller than in vivo embryos and is now widely used, even though these embryos can also be cryopreserved with the slow cooling method [183,184].
Procedures aiming at collapsing the blastocyst before vitrification through blastocoel micro-aspiration appear to improve post-thawing pregnancy rates in humans [185] as well as in horses where post-thawing pregnancy rates of embryos larger than 300µm were similar to that for embryos smaller than 300 µm [183,186]. In humans, blastocyst collapse could be routinely performed in IVF laboratories using a laser or blastocoel micro-aspiration but as with the horse, the results are controversial, and this technique involves additional handling. However, this method requires a micromanipulator, which is too expensive and too complicated to become widespread in the equine embryo transfer industry. Very recently good pregnancy rates were obtained with >300 µm embryos that were vitrified after manual blastocoel puncture [187]. If this is confirmed, this technique can be used on a larger scale and, unlike humans and other ruminants, the freezing of equine embryos may develop rapidly.
In humans, the cryopreservation of embryos has raised ethical, moral, and legal questions. In addition, the embryos belong to the couple, which can be problematic in case of separation. Therefore, some countries such as Italy have widely developed oocyte cryopreservation as an alternative to previous ban on freezing embryos.
Oocytes are more sensitive to freezing and thawing procedures and the use of vitrification has considerably improved the results (reviewed in [181]), allowing ART center to largely proposed oocyte cryopreservation in fertility preservation programs. Despite enormous progress made in vitrification techniques, oocytes remain the cells mostly exposed to damage in the freezing/thawing process when compared to embryos and spermatozoa [188,189]. For example, the volume to surface ratio of the oocyte is greater than other cells, thus complicating the dehydration process. Moreover, membrane characteristics and permeability differences appear to make the oocyte more sensitive to chilling injury. It is well known that oocyte cryopreservation induces premature cortical granule release, causing zona hardening that interferes with fertilization, requiring the use of ICSI. Additionally, due to the high rates of oocyte derived human aneuploidy, alterations to the oocyte meiotic spindle in response to low temperatures from cryopreservation are concerning with regard to potential impact on chromosome remodeling [190]. In horses, the first foals born after oocyte cryopreservation were reported in 2002 [191], but only 14% of pregnancy rates were obtained after oocyte transfer in the oviduct of recipient mares. More recently, after oocyte vitrification, only a few blastocysts were obtained and resulted in an even smaller number of foals [192,193]. It has been shown that, in equine, vitrification could lead to aberrant spindle configuration, leading to poor chromosome alignment [192,194]. As OPU is well established in horses and oocyte shipment is in general necessary, the cryopreservation of equine oocytes before ICSI is a promising technique which requires more studies. Indeed, the first foal born after oocyte vitrification and ICSI has been reported recently [195].
4. Effects of Maternal Environment in Equine and Human
To date, in humans, it has been clearly admitted that advanced maternal age, obesity, excessive sport practice, and in contrast, a sedentary lifestyle, alcohol, and tobacco, have a clearly negative effect on fertility with an extended time to pregnancy. Some maternal environments also are problematic in horses, such as maternal aging, obesity, or excessive sport.
4.1. Effects of Maternal Age
Age is a major problem in humans, as the delay for conception gradually increases with time for women, for whom, aging is a challenge for conception. Horses are facing the same issues about maternal aging. Indeed, as women who have a professional career, mares have a sporting career before producing foals. As in humans, fertility decreases with age [196,197]. It was calculated that if a 10% reduction in fertility was used as a threshold for keeping a mare in breeding programs, mares older than nine years should not be conserved [197]. Thus, the choice of ART in both species is related to maternal aging. In the equine industry, OPU–ICSI–ET is essentially used in older mares, with embryo transfer in younger, healthy recipient mares so as to reduce age-related potential effects [198].
In horses, reproductive tract dysfunction [199], reduced ovarian reserve [89,200], and decreased oocyte quality [201,202,203,204] have been well identified as age-related factors affecting fertility. Reproductive tract degeneration occurs gradually as mares grow older with oviduct lumps [81] and uterine cysts being frequently observed in old mares. Both are thought to impair oocyte and embryo movement and therefore reduce fertility. In relation to mares’ parity, severe endometrial fibrosis is also observed [199,205,206], together with increased vessel elastosis [207,208] and several deleterious histological modifications [199,209].
In humans, 50% of fertilized embryos do not implant and most of the implantation failures are mainly due to aneuploidy and these abnormalities are predominantly oocyte-related, since between 20% and 30% of human oocytes have defects of this type [210] and maternal aging is the only etiologic factor unequivocally linked to embryo aneuploidy [211]. In contrast, in horses, in vivo fertilization rates are >90% regardless of maternal age but when in vivo embryos from old mares are transferred to young recipient mares, pregnancy rates are lower [67,212]. Oocyte quality is negatively associated with maternal age [213,214] and fewer oocytes reach the second metaphase during in vitro maturation when mares are old [215]. Nevertheless, similar mechanisms are altered by maternal aging: aneuploidy, with age-related chromosome misalignment on the metaphase II spindle [201], is the most visible biological process affected by the age-related oocyte quality drop in humans and in horses [216,217]. In both species, oocyte aneuploidy is mainly due to a premature separation of sister chromatids at the end of the prophase I [210,216,218]. Moreover, for both species and unlike rodents, the meiotic spindle assembles in a slow and unstable way and in equine, maternal age predisposes to more spindle instability, which can partly explain segregation errors [219].
Recently, an impact of age has been reported on single human oocyte transcriptome [220]. In particular, the expression of genes related to mitochondrial activity was reduced [221]. This provides arguments to use mitochondrial transfer to improve IVF pregnancy rates in elderly women [222], even if more data are necessary before an eventual implementation. Globally, age could impact all proteins [223,224] and aneuploidy is only the most accessible defect detected using conventional technologies. In horses, to the author’s knowledge, high throughput analysis of the oocyte is not yet available. The expression of 48 genes was analyzed in cumulus–oocyte complexes according to maternal age and showed an age-related differential expression associated with lower quality and reduced developmental competency in oocytes from old mares [204]. In vivo, the expression of a limited number of genes required for follicular and oocyte maturation was studied in the cumulus cells and the oocyte, showing asynchronous expression in old compared to young mares [202]. The addition of follicular fluid from young or old mares to in vitro oocyte maturation medium, however, did not influence oocytes maturation nor developmental competence [225]. Like humans, mitochondrial function is also perturbed by mares’ age. Indeed, a temporal reduction of mtDNA copy numbers was described in oocytes from old mares during in vivo [202] and in vitro [203] maturation. As compared to younger controls, the oocyte mitochondria of old mares (>12 y.o.) were more swollen and had lost transverse cristae after in vitro maturation, suggesting mitochondrial degeneration during the IVM process [203]. The age-related negative effects observed in oocytes are also found in embryos. Mitochondrial DNA is less abundant in in vitro produced blastocysts when the oocyte has been collected in an old mare [226]. In addition, one study, using RNA sequencing of equine day 8 blastocysts, showed that maternal age disturbs mitosis, translation and transcription, cell signaling and adhesion, mitochondrial function, and cell fate commitment [227].
Finally, age has been associated with de novo mutations. First described in men, for whom an increased incidence of achondroplasia has been observed according to age [228]), there is growing evidence that it may concern both sexes [229]. With age, an accumulation of de novo mutations occurred at spermatogonial steps due to the continual renewal of the stem cells pool by mitosis [230]. Such mechanisms also explain the increased incidence of cancer according to age. In women, increased incidence of autism and schizophrenia has been observed in offspring born to elderly parents, pathologies which are known to be associated with de novo mutation [229]. So far, there is no such study in horses.
4.2. Effects of Maternal Obesity
The prevalence of obesity is currently close to 15% of the general population in humans [231], with more than half of women of reproductive age being overweight (BMI ≥ 25 kg/m2) [232]. Obesity is also a growing concern in horses, probably in relation to the fact that horses are increasingly being regarded as pets. Depending on the location and use of the horses, 2–72% are considered as overweight and 1–19% as obese [13,14,233,234,235,236], with the lowest incidence observed in athletic horses involved in competition. Associated with obesity, the equine metabolic syndrome (EMS) [237] is comparable to the human metabolic syndrome [238].
In humans, female obesity has been clearly associated with several adverse pregnancy outcomes [239], including risk factors for infertility whatever the menstrual cycles or smoking habits, with a higher risk in primiparous women [240]. This phenomenon seems to be mainly due to anovulation [241]. In contrast, in mares, obesity and insulin resistance have been associated with altered estrous cycles [15,242], reduction or loss of anestrus in winter [243,244], and increased incidence of LUF [15,242], but others did not report any difference on ovarian activity nor estrous cycle according to maternal BCS [245].
In women, decreased oocyte retrieval, reduced oocyte quality, and reduced rates of pre-implantation embryo development are generally observed [246] as well as poorer IVF outcomes and a decreased probability of live birth following IVF, worsened when associated with polycystic ovary syndrome [247]. In addition, when higher doses of gonadotropins are used, there is an increased risk of cycle cancellation or miscarriage [246]. Recently, using proteomic analysis, it was shown that granulosa cells mitochondria were damaged and that the endoplasmic reticulum stress response was accompanied by dysregulated hormonal synthesis [248]. These alterations may be related to the high free fatty acid and triglyceride levels detected in human follicular fluid [248] and altogether indicate a poor oocyte quality.
Even though fertility does not seem to be very affected, mainly due to the relative lack of extreme obesity in horses, the obese mare shares a lot of similarities with the obese woman. Insulin resistance, hyperinsulinemia [15,242,249] and several hormonal changes, such as increased plasma leptin, insulin-like growth factor 1, prolactin, and decreased plasmatic thyroxine, are related to obesity in mares [15,243,249]. Leptin has been suggested to support luteal establishment in a dose-dependent way in equine [116]. Moreover, as in humans, equine obesity is associated with increased inflammatory factors [250]. The uterus appears to secrete more inflammatory molecules before implantation when mares are insulin resistant compared to control mares [251]. In addition, the composition of follicular fluid in metabolic hormones and cytokines of follicles from mares with EMS or only obese are altered [252,253]. EMS and obesity also only alter expression of genes related to lipid homeostasis, endoplasmic reticulum stress, and mitochondrial function in granulosa and cumulus cells [252,253]. Moreover, the lipid composition of oocytes and embryos is perturbed by maternal obesity [249,253], suggesting reduced oocyte quality. In embryos, the expression of genes related to inflammation, lipid homeostasis, endoplasmic reticulum, as well as oxidative and mitochondrial stress is also altered by maternal obesity [249].
4.3. Effects of Maternal Excess Sport
Underweight (body mass index (BMI) below 18.5 kg/m2) is associated with an increased risk of anovulatory infertility.
In humans, the main aetiologies are (1) eating disorders that impair female fecundity due to the ovulatory dysfunction and a reduction in sexual activity, (2) energy deficiency mainly due to excess sport practice and that mainly concerns female athletes, (3) lipodystrophies, a heterogeneous group of rare disorders, characterized by selective loss of adipose tissue in the absence of nutritional deprivation or catabolic state, (4) starvation and undernutrition due to the self-imposed dietary restriction in developed countries, or malnutrition in developing countries, and (5) a few chronic systemic diseases [254]. Regarding physically active women, a medical triad is observed associating low energy availability with or without disordered eating, menstrual dysfunction, and low bone density. Health is a prerequisite for optimal performance, and menstruation is often considered as a problem for most of them, with either a negative or neutral impact on performance [255]. Although a systematic review and meta-analysis indicated that exercise performance might be trivially reduced during the early follicular phase, they concluded that a personalized approach should be taken, based on everyone’s response to exercise performance across the menstrual cycle. So, even if there is no clear evidence for a relation between performance and menstrual cycle, hormonal medication for both contraception and to manipulate the menstrual cycle is very commonly used [256]. Based on a cohort of elite female athletes, oligomenorrhea/amenorrhea has been reported to be higher in athletes without hormonal contraception. Stress fractures and iron deficiency, common for these women, are associated with oligomenorrhoea/amenorrhea/menorrhagia and eating disorders. In addition, in this cohort, 15% of these women were engaged in disordered eating practices in order to conform to gender ideals [257]. Whatever the consequence of such practice, a recent study, comparing a few elite athletes to active controls, reported that regularly physically active (>150 min per week) elite athletes get pregnant easily and deliver healthy babies [258].
Apart from being underweight, associated with anovulation and metabolic disorders, it seems that elite female athletes are not particularly infertile. However, in general, it is necessary to stop training and competing for a long period to have a child, and many of them decide to delay pregnancy. Oocyte preservation could be an opportunity for these women.
In the equine industry, ART, especially embryo transfer, is very successful in competing horses because it enables breeders to produce foals when mares are still performing. Effects of sport on reproductive outcomes in horses have been related to a higher internal temperature in exercising mares which can be deleterious for reproduction [259]. Indeed, altered ovarian follicle as well as endocrine (cortisol and LH) dynamics are observed in exercising mares [259,260]. Effects of sports activity on embryo quality and recovery rates are controversial as reduced embryo recovery rates [259,261], with poor embryo quality [259] have been reported by some in exercising mares while others did not observe any deleterious effect [262]. The latter observation could be due to better cycle monitoring that has been shown to improve embryo recovery efficiency in exercising mares [263]. Depending on breed, racing after insemination/mating has been shown to be deleterious for foal production [264], but moderate exercise did not influence pregnancy outcomes [265]. Furthermore, one study showed that retired performance mares have a much higher incidence of glandular differentiation disorders related to ovarian dysfunction [266] compared to non performing controls.
5. Where Is the Mare a Good Model for ART in Humans?
Overall comparisons between the physiological and ART specificities of woman and mare are shown in Table 1. Contrary to laboratory models, the horse is a long lived monocotous animal. Its high economical and sentimental value to humans justifies its use in reproduction until old age and the investment in state-of-the-art ART to overcome infertility and to increase foal production. As seen in Table 1, there are many similarities but also large differences in the physiology, ART and effects of environment between humans and horses. Yet, the horse may be an excellent model, offering possibilities not enabled by other models to study ART for humans. Its large size also enables repeated sampling that is not allowed by smaller models. Alternatively, the human may be also a very valuable source of inspiration for scientists and veterinarians working with horses.
Table 1. Summary of similarities and differences in periconceptional physiology and ART procedures between the woman and the mare. LUF: Luteinized Unovulatory Follicle; OPU: Ovum Pick-up; PCOS: Polycystic ovary syndrome.
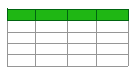
5.1. Developmental Origins of Health and Disease
In the past, ART has been associated in other species with adverse effects during pregnancy, e.g., with the onset of large offspring syndrome in cattle [267]. In addition, periconceptional environment and ART are now known to affect long term offspring phenotype through the establishment of epigenetic marks in the early stages of development [268]. Although valuable information on these effects is collected using rodent and other models [147], large monocotous animals with a long lifespan supported by a sports industry and for which ART is used with similar objectives as in humans can bring invaluable information on the long-term effects of ART. Using horses of known genetic background, health and performance comparisons between offspring produced by natural mating, artificial insemination or ART could be organized, whether prospectively or retrospectively through epidemiological studies, as performance and genealogy is recorded by studbooks of national organizations such as the French institute of horse and horse riding (IFCE) in France. The horse, with a completely sequenced genome (×6.8 in 2021) [269] is also a valuable model to look at differences in gene expression in embryos. Moreover, its size enables embryo bisection and subsequent differential analysis of gene expression in the inner cell mass and the trophoblast [227], which could bring important insight into mechanisms.
5.2. Follicular Cycle
With a very long follicular phase, during which the mare accepts breeding, the mare is unique among domestic animals. A long follicular phase is also present in women. Thus, the mare could be a very good model for studying the regulation of follicular growth. Because of the particular structure of the equine ovary and its ovulation fossa, and because of the large follicular size, experimental sampling of follicular fluids throughout the cycle can be performed to study follicular maturation, without rupturing the follicle [270]. Complete removal of follicular fluid from a preovulatory follicle was used to demonstrate that follicular fluid was not necessary for ovulation [271]. The same approach enables the injection into the follicle of experimental compounds, immature oocytes to perform an “ex vivo maturation” [272], or spermatozoa. In addition, the destruction of follicles through ultrasound-guided follicular puncture starts a new follicular wave and enables an exact timing of follicular growth. Finally, with the occurrence of LUF in both equine and human, the mare has thus been suggested as a potential model for investigating anovulatory infertility in women [273].
5.3. Oocyte Retention in the Oviduct
The mare is unique in that unfertilized oocytes remain in the oviduct. Given that most ectopic pregnancies in women occur in the oviduct [274], it might be interesting to use the mare model to study factor involved leading to the embryo-driven movement in the oviduct. For example, topical PGE2 application to the oviduct has been shown to induce oviductal patency [275].
5.4. Oocyte In Vitro Maturation (IVM)
As mentioned above, IVM is only used in a few clinical situations in humans and thus, IVM conditions have not been improved for many years. Since IVM is almost compulsory in the equine species, the horse could be a good model for humans to work on IVM conditions.
5.5. Effects of Environment: Aging, Obesity and Excess Sport
The horse is a species where animals are bred at an old age, with breeders that continue breeding as long as they can produce a foal from their favorite mare(s). It is thus common to see nulliparous mares (mares that did not previously foal, also known as maiden mares) bred at old age. Indeed, nulliparous mares older than 10 years of age have been shown to represent 20.5% of Finn horse and 15.5% of Standardbred bred mares in Finland [276]. Conversely, many old mares had had a large number of foals. Thus, the mare has been proposed as a model for oocyte aging, especially since similar mechanisms are involved in both species, including a high incidence of aneuploidy and mitochondrial defects [10,213].
As mentioned in Section 4.2, excess BMI (overweight and obesity) is frequent in horses, although morbid obesity is extremely rare. The incidence of the equine metabolic syndrome also increases in the equine population, as well as in the human population. Deleterious effects of excess body weight on oocyte quality, follicular cycles, and reproductive tract inflammation are shared between the two species. In addition, obesity and the metabolic syndrome are often associated with aging in both species.
Since progress in understanding and preventing the effects of aging and obesity on oocyte would benefit both species, research in this area could be co-funded by medical and veterinary research.
In terms of excess sport, the intense training of thoroughbred mares in their early age and their very thin body condition at this stage could also be used to model effects of excess sport in young women. In particular, long-term consequences on reproductive outcomes and epigenetic effects could be followed up in horses.
5.6. Where Is the Mare Definitely Not a Good Model for ART in Humans?
In terms of physiology, the first birth of IVF foals [142] was never reproduced in horses and only ICSI is currently used for fertilization of equine oocytes [111]. Thus, the horse cannot be used as a model for human IVF. In addition, the horse uterus is not able to sustain twin pregnancies, in contrast to humans.
As mentioned before, PCOS is not a spontaneous pathology known in horses, and thus horses cannot be used as models for this human disease, unless it could be induced by exogenous treatment or genetic selection as already done in other species [277,278].
5.7. Where Can the Woman Help the Mare?
Many ARTs are used in women that are not mastered in horses. In particular, embryo and oocyte cryopreservation need to be improved in mares and experience from human biologists is certainly of great help.
6. Conclusions
In conclusion, although there are large differences between horses and humans in terms of reproductive anatomy, physiology, and ART, both species share infertility concerns due to ageing and obesity. Some techniques have been mastered in humans but not in horses, such as superovulation, as well as embryo and oocyte cryopreservation, whereas others are more advanced in horses, such as IVM. Thus, reciprocal knowledge could be gained through the comparative study of ART and infertility treatments both in women and mares, even though the horse could not be used as a single model for human ART.
Women and mares both ovulate a metaphase II oocyte (1). In both species, once fertilization is achieved, the oocyte resumes its meiosis (2). Extrusion of the second polar body happens quickly after fertilization and is followed by the formation of the female and male pronuclei (PN). In women, PN fading is observable 23 ± 1 h post insemination (HPI). Then, both pronuclei merge to form the nucleus. By 27 ± 1 hpi in women and 24 h in mares, the first cleavage occurs (3). The second division takes place over the next 24 h in both species (4) and the 8-cell stage is reached within 3 days post fertilization (5). In women, this is the last developmental stage in the fallopian tube. During the next day, a morula with at least 16 cells is formed (6). In horses, this is the last stage of development in the oviduct. The morula produces prostaglandin E2, that relaxes the muscles of the isthmus of the oviduct (utero–tubal junction) and enable the passage of the embryo into the uterus. Development to the blastocyst stage (6) is a little longer in horses than in humans (reached at 116 ± 2 HPI in humans and 7 days in horses). This is the last common developmental stage between the two species.
Author Contributions
Conceptualization, P.C.-P., E.D., F.V., E.P.; outline: P.C.-P., E.D., A.B., M.P., F.V.; writing—original draft preparation: A.B., E.D., P.C.-P., M.P., F.V.; writing—review and editing: A.B., E.D., P.C.-P., E.P., J.M.A., M.P.; visualization, E.D.; supervision, P.C.-P., F.V., J.M.A. All authors have read and agreed to the published version of the manuscript.
Funding
No funding was obtained for this article.
Data Availability Statement
Data sharing not applicable.
Conflicts of Interest
The authors declare no conflict of interest.
ReferencesOldenbroek, K.; van der Waaij, L. Introduction to animal breeding. Textbook Animal Breeding and Genetics for BSc Students; Centre for Genetic Resources The Netherlands and Animal Breeding and Genomics Centre, 2015. Available online: https://wiki.groenkennisnet.nl/display/TAB/ (accessed on 22 June 2021).
Viana, J.H. 2019 Statistics of embryo production and transfer in domestic farm animals. Divergent Trends IVD IVP Embryos. Embryo Technol. Newsl. 2020, 38, 7–26. [Google Scholar]
Palmer, E.; Chavatte-Palmer, P. Contribution of reproduction management and technologies to genetic progress in horse breeding. J. Equine Vet. Sci. 2020, 103016. [Google Scholar] [CrossRef] [PubMed]
United Nations, Department of Economic and Social Affairs, Population Division. World Fertility Report 2015-Highlights; United Nations, 2017. Available online: https://www.un.org/development/desa/pd/sites/www.un.org.development.desa.pd/files/files/documents/2020/Feb/un_2015_worldfertilityreport_highlights.pdf (accessed on 22 June 2021).
Attali, E.; Yogev, Y. The impact of advanced maternal age on pregnancy outcome. Best Pract. Res. Clin. Obstet. Gynaecol. 2021, 70, 2–9. [Google Scholar] [CrossRef] [PubMed]
Hanna, C.W.; Demond, H.; Kelsey, G. Epigenetic regulation in development: Is the mouse a good model for the human? Hum. Reprod. Update 2018, 24, 556–576. [Google Scholar] [CrossRef] [PubMed]
Fischer, B.; Chavatte-Palmer, P.; Viebahn, C.; Navarrete Santos, A.; Duranthon, V. Rabbit as a reproductive model for human health. Reproduction 2012, 144, 1–10. [Google Scholar] [CrossRef] [PubMed]
Anckaert, E.; Fair, T. DNA methylation reprogramming during oogenesis and interference by reproductive technologies: Studies in mouse and bovine models. Reprod. Fertil. Dev. 2015, 27, 739. [Google Scholar] [CrossRef]
Carnevale, E.M.; Catandi, G.D.; Fresa, K. Equine aging and the oocyte: A potential model for reproductive aging in women. J. Equine Vet. Sci. 2020, 89, 103022. [Google Scholar] [CrossRef]
Carnevale, E.M. The mare model for follicular maturation and reproductive aging in the woman. Theriogenology2008, 69, 23–30. [Google Scholar] [CrossRef]
Morris, L.H.; Maclellan, L.J. Factors affecting in vitro blastocyst production after intracytoplasmic sperm injection (ICSI). J. Equine Vet. Sci. 2020, 89, 103055. [Google Scholar] [CrossRef]
Allen, W.R.; Wilsher, S. Half a century of equine reproduction research and application: A veterinary tour de force. Equine. Vet. J. 2018, 50, 10–21. [Google Scholar] [CrossRef]
Giles, S.L.; Rands, S.A.; Nicol, C.J.; Harris, P.A. Obesity prevalence and associated risk factors in outdoor living domestic horses and ponies. PeerJ 2014, 2, e299. [Google Scholar] [CrossRef]
Robin, C.A.; Ireland, J.L.; Wylie, C.E.; Collins, S.N.; Verheyen, K.L.P.; Newton, J.R. Prevalence of and risk factors for equine obesity in Great Britain based on owner-reported body condition scores: Prevalence of and risk factors for equine obesity in Great Britain. Equine Vet. J. 2015, 47, 196–201. [Google Scholar] [CrossRef] [PubMed]
Vick, M.M.; Sessions, D.R.; Murphy, B.A.; Kennedy, E.L.; Reedy, S.E.; Fitzgerald, B.P. Obesity is associated with altered metabolic and reproductive activity in the mare: Effects of metformin on insulin sensitivity and reproductive cyclicity. Reprod. Fertil. Dev. 2006, 18, 609. [Google Scholar] [CrossRef] [PubMed]
Masko, M.; Domino, M.; Skierbiszewska, K.; Zdrojkowski, Ł.; Jasinski, T.; Gajewski, Z. Monitoring of the mare during the perinatal period at the clinic and in the stable. Equine Vet. Educ. 2020, 32, 654–663. [Google Scholar] [CrossRef]
Monniaux, D.; Clément, F.; Dalbiès-Tran, R.; Estienne, A.; Fabre, S.; Mansanet, C.; Monget, P. The Ovarian Reserve of Primordial Follicles and the Dynamic Reserve of Antral Growing Follicles: What Is the Link? Biol. Reprod. 2014, 90. [Google Scholar] [CrossRef] [PubMed]
Haadsma, M.L.; Groen, H.; Fidler, V.; Bukman, A.; Roeloffzen, E.M.A.; Groenewoud, E.R.; Broekmans, F.J.M.; Heineman, M.J.; Hoek, A. The predictive value of ovarian reserve tests for spontaneous pregnancy in subfertile ovulatory women. Hum. Reprod. 2008, 23, 1800–1807. [Google Scholar] [CrossRef]
Driancourt, M.A.; Paris, A.; Roux, C.; Mariana, J.C.; Palmer, E. Ovarian follicular populations in pony and saddle-type mares. Reprod. Nutr. Dévelop. 1982, 22, 1035–1047. [Google Scholar] [CrossRef]
Ginther, O.J. Reproductive Biology of the Mare: Basic And Applied Aspects, 2nd ed.; Equiservices: Cross-Plains, WI, USA, 1992. [Google Scholar]
Messinis, I.E. From menarche to regular menstruation: Endocrinological background. Ann. N.Y. Acad. Sci. 2006, 1092, 49–56. [Google Scholar] [CrossRef]
Burkhardt, J. Transition from anoestrus in the mare and the effects of artificial lighting. J. Agric. Sci. 1947, 37, 64–68. [Google Scholar] [CrossRef]
Nishikawa, Y. Studies on Reproduction in Horses. Singularity and Artificial Control in Reproductive Phenomena; Japan Racing Association: Tokyo, Japan, 1959. [Google Scholar]
Donadeu, F.; Pedersen, H. Follicle development in mares. Reprod. Domest. Anim. 2008, 43, 224–231. [Google Scholar] [CrossRef]
Craig, J. Gonadotropin and intra-ovarian signals regulating follicle development and atresia: The delicate balance between life and death. Front. Biosci. 2007, 12, 3628. [Google Scholar] [CrossRef]
Gougeon, A. Qualitative changes in medium and large antral follicles in the human ovary during the menstrual cycle. Ann. Biol. Anim. Bioch. Biophys. 1979, 19, 1461–1468. [Google Scholar] [CrossRef]
Hansen, K.R.; Knowlton, N.S.; Thyer, A.C.; Charleston, J.S.; Soules, M.R.; Klein, N.A. A new model of reproductive aging: The decline in ovarian non-growing follicle number from birth to menopause. Hum. Reprod.2008, 23, 699–708. [Google Scholar] [CrossRef]
Gougeon, A. Dynamics of follicular growth in the human: A model from preliminary results. Hum. Reprod. 1986, 1, 81–87. [Google Scholar] [CrossRef]
Ginther, O.J.; Gastal, E.L.; Gastal, M.O.; Bergfelt, D.R.; Baerwald, A.R.; Pierson, R.A. Comparative study of the dynamics of follicular waves in Mares and women. Biol. Reprod. 2004, 71, 1195–1201. [Google Scholar] [CrossRef]
Baerwald, A.R.; Adams, G.P.; Pierson, R.A. Characterization of ovarian follicular wave dynamics in women. Biol. Reprod. 2003, 69, 1023–1031. [Google Scholar] [CrossRef] [PubMed]
Ginther, O.J. The mare: A 1000-pound guinea pig for study of the ovulatory follicular wave in women. Theriogenology 2012, 77, 818–828. [Google Scholar] [CrossRef] [PubMed]
Gougeon, A. Dynamics of follicle development in the human ovary. In Polycystic Ovary Syndrome; Chang, R.J., Ed.; Springer: New York, NY, USA, 1996; pp. 21–36. [Google Scholar] [CrossRef]
Rapuri, P.B.; Gallagher, J.C.; Haynatzki, G. Endogenous levels of serum estradiol and sex hormone binding globulin determine bone mineral density, bone remodeling, the rate of bone loss, and response to treatment with estrogen in elderly women. J. Clin. Endocrinol. Metab. 2004, 89, 4954–4962. [Google Scholar] [CrossRef] [PubMed]
Sato, K.; Miyake, M.; Yoshikawa, T.; Kambegawa, A. Studies on serum oestrogen and progesterone levels during the oestrous cycle and early pregnancy in mares. Equine Vet. J. 1977, 9, 57–60. [Google Scholar] [CrossRef]
Palmer, E. New results on follicular growth and ovulation in the mare. In Follicular Growth and Ovulation Rate in Farm Animals; Springer: Dordrecht, The Netherlands, 1987; pp. 237–255. [Google Scholar]
Lebedeva, L.F.; Solodova, E.V. Technological approaches to the problem of double ovulation and twin pregnancies in mares. IOP Conf. Ser. Earth Environ. Sci. 2021, 624, 012036. [Google Scholar] [CrossRef]
Morel, M.C.G.D.; Newcombe, J.R.; Swindlehurst, J.C. The effect of age on multiple ovulation rates, multiple pregnancy rates and embryonic vesicle diameter in the mare. Theriogenology 2005, 63, 2482–2493. [Google Scholar] [CrossRef] [PubMed]
Ginther, O.J. Twinning in mares: A review of recent studies. J. Equine Vet. Sci. 1982, 2, 127–135. [Google Scholar] [CrossRef]
Santana, D.S.; Souza, R.T.; Surita, F.G.; Argenton, J.L.; Silva, C.M.; Cecatti, J.G. Twin pregnancy in Brazil: A Profile analysis exploring population information from the national birth e-registry on live births. BioMed Res. Int.2018, 2018, e9189648. [Google Scholar] [CrossRef] [PubMed]
Levasseur, M.-C. Utero-ovarian relationships in placental mammals: Role of uterus and embryo in the regulation of progesterone secretion by the corpus luteum. Rev. Reprod. Nutr. Dévelop. 1983, 23, 793–816. [Google Scholar] [CrossRef] [PubMed]
Stouffer, R.L.; Bishop, C.V.; Bogan, R.L.; Xu, F.; Hennebold, J.D. Endocrine and local control of the primate corpus luteum. Reprod. Biol. 2013, 13, 259–271. [Google Scholar] [CrossRef]
Ginther, O.J. A 40-year odyssey into the mysteries of equine luteolysis. Theriogenology 2009, 72, 591–598. [Google Scholar] [CrossRef]
Wallach, E.E.; Katz, E. The luteinized unruptured follicle other ovulatory dysfunctions. Fertil. Steril. 1988, 50, 839–850. [Google Scholar] [CrossRef]
Check, J.H. Ovulation disorders: Part II. Anovulation associated with normal estrogen. Clin. Exp. Obstet. Gynecol. 2007, 34, 69–72. [Google Scholar]
Kaiser, B.; Koene, M.; Swagemakers, J.; Bader, H.; Hoppen, H. Diagnosis, therapy and endocrinologic parameters of persistent follicles in mares in comparison with preovulatory follicles. Tierarztl. Prax. Ausg. G. Grosstiere/Nutztiere 1999, 27, 180–186. [Google Scholar]
Ginther, O.J.; Gastal, E.L.; Gastal, M.O.; Beg, M.A. Incidence, endocrinology, vascularity, and morphology of hemorrhagic anovulatory follicles in Mares. J. Equine Vet. Sci. 2007, 27, 130–139. [Google Scholar] [CrossRef]
Cuervo-Arango, J.; Newcombe, J.R. The effect of cloprostenol on the incidence of multiple ovulation and anovulatory hemorrhagic follicles in two mares: A case report. J. Equine Vet. Sci. 2009, 29, 533–539. [Google Scholar] [CrossRef]
Hamilton, C.J.C.M.; Evers, J.L.H.; Hoogland, H.J. Ovulatory disorders and inflammatory adnexal damage: A neglected cause of the failure of fertility microsurgery. BJOG Int. J. OG 1986, 93, 282–284. [Google Scholar] [CrossRef]
Qublan, H.; Amarin, Z.; Nawasreh, M.; Diab, F.; Malkawi, S.; Al-Ahmad, N.; Balawneh, M. Luteinized unruptured follicle syndrome: Incidence and recurrence rate in infertile women with unexplained infertility undergoing intrauterine insemination. Hum. Reprod. 2006, 21, 2110–2113. [Google Scholar] [CrossRef] [PubMed]
Kerin, J.F.; Kirby, C.; Morris, D.; McEvoy, M.; Ward, B.; Cox, L.W. Incidence of the luteinized unruptured follicle phenomenon in cycling women**Supported in part by National Health and Medical Research Council (NH&MRC) grant 810549. Fertil. Steril. 1983, 40, 620–626. [Google Scholar] [CrossRef] [PubMed]
Dal, J.; Vural, B.; Caliskan, E.; Ozkan, S.; Yucesoy, I. Power Doppler ultrasound studies of ovarian, uterine, and endometrial blood flow in regularly menstruating women with respect to luteal phase defects. Fertil. Steril. 2005, 84, 224–227. [Google Scholar] [CrossRef]
Kaya, H.; Oral, B. Effect of ovarian involvement on the frequency of luteinized unruptured follicle in endometriosis. Gynecol. Obs. Investig. 1999, 48, 123–126. [Google Scholar] [CrossRef]
Koninckx, P.R.; Brosens, I.A. The luteinized unruptured follicle syndrome. Fertil. Steril. 1983, 39, 249–250. [Google Scholar] [CrossRef]
Kugu, K.; Taketani, Y.; Kohda, K.; Mizuno, M. Exaggerated prolactin response to thyrotropin-releasing hormone in infertile women with the luteinized unruptured follicle syndrome. Arch. Gynecol. Obstet. 1991, 249, 27–31. [Google Scholar] [CrossRef]
Lefranc, A.-C.; Allen, W.R. Incidence and morphology of anovulatory haemorrhagic follicles in the mare. Pferdeheilkunde 2003, 19, 611–612. [Google Scholar] [CrossRef]
Ginther, O.J.; Gastal, M.O.; Gastal, E.L.; Jacob, J.C.; Beg, M.A. Induction of haemorrhagic anovulatory follicles in mares. Reprod. Fertil. Dev. 2008, 20, 947. [Google Scholar] [CrossRef] [PubMed]
Gastal, E.L.; Gastal, M.O.; Ginther, O.J. The suitability of echotexture characteristics of the follicular wall for identifying the optimal breeding day in mares. Theriogenology 1998, 50, 1025–1038. [Google Scholar] [CrossRef]
Clewe, T.H.; Mastroianni, L.J. Mechanisms of ovum pickup. I. functional capacity of rabbit oviducts ligated near the fimbria. Obstet. Gynecol. Surv. 1958, 13, 551–552. [Google Scholar] [CrossRef]
Corpa, J.M. Ectopic pregnancy in animals and humans. Reproduction 2006, 131, 631–640. [Google Scholar] [CrossRef] [PubMed]
Enders, A.C.; Liu, I.K.M.; Bowers, J.; Lantz, K.C.; Schlafke, S.; Suarez, S. The ovulated ovum of the horse: Cytology of nonfertilized ova to pronuclear stage ova1. Biol. Reprod. 1987, 37, 453–466. [Google Scholar] [CrossRef]
Betteridge, K.J.; Eaglesome, M.D.; Mitchellt, D.; Flood, P.F.; Beriault, R. Development of horse embryos up to twenty two days after ovulation: Observations on fresh specimens. J. Anat. 1982, 135, 191–209. [Google Scholar]
Flood, P.F.; Betteridge, K.J.; Diocee, M.S. Transmission electron microscopy of horse embryos 3-16 days after ovulation. J. Reprod. Fertil. Suppl. 1982, 32, 319–327. [Google Scholar] [PubMed]
Balaban, B.; Brison, D.; Calderon, G.; Catt, J.; Conaghan, J.; Cowan, L.; Ebner, T.; Gardner, D.; Hardarson, T. Alpha scientists in reproductive medicine and eshre special interest group of embryology. The istanbul consensus workshop on embryo assessment: Proceedings of an expert meeting. Hum. Reprod. 2011, 26, 1270–1283. [Google Scholar] [CrossRef]
Cruz, M.; Garrido, N.; Herrero, J.; Pérez-Cano, I.; Muñoz, M.; Meseguer, M. Timing of cell division in human cleavage-stage embryos is linked with blastocyst formation and quality. Reprod. Biomed. Online 2012, 25, 371–381. [Google Scholar] [CrossRef] [PubMed]
Milewski, R.; Ajduk, A. Time-lapse imaging of cleavage divisions in embryo quality assessment. Reproduction2017, 154, R37–R53. [Google Scholar] [CrossRef]
Grøndahl, C.; Hyttel, P. Nucleologenesis and ribonucleic acid synthesis in preimplantation equine embryos1. Biol. Reprod. 1996, 55, 769–774. [Google Scholar] [CrossRef] [PubMed]
McKinnon, A.O.; Squires, E.L. Morphologic assessment of the equine embryo. J. Am. Vet. Med Assoc. 1988, 192, 401–406. [Google Scholar]
Lane, M.; O’Donovan, M.K.; Squires, E.L.; Seidel, G.E.; Gardner, D.K. Assessment of metabolism of equine morulae and blastocysts. Mol. Reprod Dev. 2001, 59, 33–37. [Google Scholar] [CrossRef]
Ball, B.A.; Little, T.V.; Weber, J.A.; Woods, G.L. Survival of Day-4 embryos from young, normal mares and aged, subfertile mares after transfer to normal recipient mares. Reproduction 1989, 85, 187–194. [Google Scholar] [CrossRef]
Enders, A.C.; Schlafke, S.; Lantz, K.C.; Liu, I.K.M. Endoderm cells of the equine yolk sac from Day 7 until formation of the definitive yolk sac placenta. Equine Vet. J. 1993, 25, 3–9. [Google Scholar] [CrossRef]
Motiei, M.; Vaculikova, K.; Cela, A.; Tvrdonova, K.; Khalili, R.; Rumpik, D.; Rumpikova, T.; Glatz, Z.; Saha, T. Non-invasive human embryo metabolic assessment as a developmental criterion. JCM 2020, 9, 4094. [Google Scholar] [CrossRef]
Uyar, A.; Seli, E. Metabolomic assessment of embryo viability. Semin. Reprod. Med. 2014, 32, 141–152. [Google Scholar] [CrossRef] [PubMed]
Gilbert, S.F.; Gilbert, S.F. Developmental Biology, 6th ed.; Sinauer Associates: Sunderland, MA, USA, 2000. [Google Scholar]
Battut, I.; Colchen, S.; Fieni, F.; Tainturier, D.; Bruyas, J.-F. Success rates when attempting to nonsurgically collect equine embryos at 144, 156 or 168 hours after ovulation. Equine Vet. J. 1998, 29, 60–62. [Google Scholar] [CrossRef] [PubMed]
Freeman, D.A.; Woods, G.L.; Vanderwall, D.K.; Weber, J.A. Embryo-initiated oviductal transport in mares. Reproduction 1992, 95, 535–538. [Google Scholar] [CrossRef] [PubMed]
Freeman, D.A.; Weber, J.A.; Geary, R.T.; Woods, G.L. Time of embryo transport through the mare oviduct. Theriogenology 1991, 36, 823–830. [Google Scholar] [CrossRef]
Weber, J.A.; Freeman, D.A.; Vanderwall, D.K.; Woods, G.L. Prostaglandin E2 secretion by oviductal transport-stage equine embryos. Biol. Reprod. 1991, 45, 540–543. [Google Scholar] [CrossRef]
Weber, J.A.; Freeman, D.A.; Vanderwall, D.K.; Woods, G.L. Prostaglandin E2 hastens oviductal transport of equine embryos1. Biol. Reprod. 1991, 45, 544–546. [Google Scholar] [CrossRef]
Weber, J.A.; Woods, G.L.; Freeman, D.A.; Vanderwall, D.K. Prostaglandin E2-specific binding to the equine oviduct. Prostaglandins 1992, 43, 61–65. [Google Scholar] [CrossRef]
Weber, J.A.; Woods, G.L.; Lichtenwalner, A.B. Relaxatory effect of prostaglandin e2 on circular smooth muscle isolated from the equine oviductal isthmus1. Biol. Reprod. 1995, 52, 125–130. [Google Scholar] [CrossRef]
Aguilar, J.J.; Woods, G.L.; Miragaya, M.H.; Olsen, L.M. Living fibroblast cells in the oviductal masses of mares. Equine Vet. J. 1997, S25, 103–108. [Google Scholar] [CrossRef] [PubMed]
Adams, C.E. Consequences of accelerated ovum transport, including a re-evaluation of Estes’ operation. Reproduction 1979, 55, 239–246. [Google Scholar] [CrossRef] [PubMed]
Beyth, Y.; Polishuk, W.Z. Ovarian implantation into the Uterus (Estes Operation): Clinical and experimental evaluation. Fertil. Steril. 1979, 32, 657–660. [Google Scholar] [CrossRef]
Neuhausser, W.M.; Vaughan, D.A.; Sakkas, D.; Hacker, M.R.; Toth, T.; Penzias, A. Non-inferiority of cleavage-stage versus blastocyst-stage embryo transfer in poor prognosis, IVF patients (PRECiSE trial): Study protocol for a randomized controlled trial. Reprod. Health 2020, 17, 16. [Google Scholar] [CrossRef]
Pasqualini, R.; Quintans, C. Clinical practice of embryo transfer. Reprod. Biomed. Online 2002, 4, 83–92. [Google Scholar] [CrossRef]
Swegen, A. Maternal recognition of pregnancy in the mare: Does it exist and why do we care? Reproduction2021, 161, R139–R155. [Google Scholar] [CrossRef]
Allen, W.R.; Wilsher, S. A Review of implantation and early placentation in the mare. Placenta 2009, 30, 1005–1015. [Google Scholar] [CrossRef] [PubMed]
Carnevale, E.M.; Bergfelt, D.R.; Ginther, O.J. Follicular activity and concentrations of FSH and LH associated with senescence in mares. Anim. Reprod. Sci. 1994, 35, 231–246. [Google Scholar] [CrossRef]
Vanderwall, D.K.; Woods, G.L.; Freeman, D.A.; Weber, J.A.; Rock, R.W.; Tester, D.F. Ovarian follicles, ovulations and progesterone concentrations in aged versus young mares. Theriogenology 1993, 40, 21–32. [Google Scholar] [CrossRef]
Steptoe, P.C.; Edwards, R.G. Birth after the reimplantation of a human embryo. Lancet 1978, 312, 366. [Google Scholar] [CrossRef]
Macklon, N.S.; Stouffer, R.L.; Giudice, L.C.; Fauser, B.C.J.M. The science behind 25 Years of ovarian stimulation for in vitro fertilization. Endocr. Rev. 2006, 27, 170–207. [Google Scholar] [CrossRef] [PubMed]
Al-Inany, H.G.; Youssef, M.A.; Ayeleke, R.O.; Brown, J.; Lam, W.S.; Broekmans, F.J. Gonadotrophin-releasing hormone antagonists for assisted reproductive technology. Cochrane Database Syst. Rev. 2016. [Google Scholar] [CrossRef]
Niederberger, C.; Pellicer, A.; Cohen, J.; Gardner, D.K.; Palermo, G.D.; O’Neill, C.L.; Chow, S.; Rosenwaks, Z.; Cobo, A.; Swain, J.E.; et al. Forty years of IVF. Fertil. Steril. 2018, 110, 185–324.e5. [Google Scholar] [CrossRef] [PubMed]
Squires, E.L.; McCue, P.M. Superovulation in mares. Anim. Reprod. Sci. 2007, 99, 1–8. [Google Scholar] [CrossRef]
Roser, J.F.; Meyers-Brown, G. Enhancing Fertility in mares: Recombinant equine gonadotropins. J. Equine Vet. Sci. 2019, 76, 6–13. [Google Scholar] [CrossRef]
Gleicher, N.; Friberg, J.; Fullan, N.; Giglia, R.; Mayden, K.; Kesky, T.; Siegel, I. Egg Retrieval for in vitro fertilisation by sonographically controlled vaginal culdocentesis. Lancet 1983, 322, 508–509. [Google Scholar] [CrossRef]
Bennett, S.J.; Waterstone, J.J.; Cheng, W.C.; Parsons, J. Complications of transvaginal ultrasound-directed follicle aspiration: A review of 2670 consecutive procedures. J. Assist. Reprod. Genet. 1993, 10, 72–77. [Google Scholar] [CrossRef]
Palmer, E.; Duchamp, G.; Bézard, J.; Magistrini, M.; King, W.A.; Betteridge, K.J. Non-surgical recovery of follicular fluid and oocytes of mares. J. Reprod. Fertil. Suppl. 1987, 35, 689–690. [Google Scholar]
Lazzari, G.; Colleoni, S.; Crotti, G.; Turini, P.; Fiorini, G.; Barandalla, M.; Landriscina, L.; Dolci, G.; Benedetti, M.; Duchi, R.; et al. Laboratory production of equine embryos. J. Equine Vet. Sci. 2020, 89, 103097. [Google Scholar] [CrossRef]
Morris, L.H.A. The development of in vitro embryo production in the horse. Equine Vet. J. 2018, 50, 712–720. [Google Scholar] [CrossRef] [PubMed]
Galli, C.; Duchi, R.; Colleoni, S.; Lagutina, I.; Lazzari, G. Ovum pick up, intracytoplasmic sperm injection and somatic cell nuclear transfer in cattle, buffalo and horses: From the research laboratory to clinical practice. Theriogenology 2014, 81, 138–151. [Google Scholar] [CrossRef]
Hawley, L.R.; Enders, A.C.; Hinrichs, K. Comparison of equine and bovine oocyte- cumulus morphology within the ovarian follicle. Biol. Reprod. Mono. 1995, 1, 243–252. [Google Scholar] [CrossRef]
Hinrichs, K. The equine oocyte: Factors affecting meiotic and developmental competence. Mol. Reprod. Dev.2010, 77, 651–661. [Google Scholar] [CrossRef] [PubMed]
Seyhan, A.; Ata, B.; Son, W.-Y.; Dahan, M.H.; Tan, S.L. Comparison of complication rates and pain scores after transvaginal ultrasound–guided oocyte pickup procedures for in vitro maturation and in vitro fertilization cycles. Fertil. Steril. 2014, 101, 705–709. [Google Scholar] [CrossRef]
Souza, M.d.C.B.d.; Souza, M.M.d.; Antunes, R.d.A.; Tamm, M.A.; Silva, J.B.d.; Mancebo, A.C.A. Bladder hematoma: A complication from an oocyte retrieval procedure. JBRA Assist. Reprod. 2019. [Google Scholar] [CrossRef]
Jacobson, C.C.; Choi, Y.-H.; Hayden, S.S.; Hinrichs, K. Recovery of mare oocytes on a fixed biweekly schedule, and resulting blastocyst formation after intracytoplasmic sperm injection. Theriogenology 2010, 73, 1116–1126. [Google Scholar] [CrossRef]
Rock, J.; Menkin, M.F. In vitro fertilization and cleavage of human ovarian eggs. Science 1944, 100, 105–107. [Google Scholar] [CrossRef]
Cha, K.Y.; Koo, J.J.; Ko, J.J.; Choi, D.H.; Han, S.Y.; Yoon, T.K. Pregnancy after in vitro fertilization of human follicular oocytes collected from nonstimulated cycles, their culture in vitro and their transfer in a donor oocyte program**Prize paper, presented at the 45th annual meeting of the american fertility society, San Francisco, California, November 11 to 16, 1989. Fertil. Steril. 1991, 55, 109–113. [Google Scholar] [CrossRef]
Trounson, A.; Wood, C.; Kausche, A. In vitro maturation and the fertilization and developmental competence of oocytes recovered from untreated polycystic ovarian patients. Fertil. Steril. 1994, 62, 353–362. [Google Scholar] [CrossRef]
Gérard, N.; Robin, E. Cellular and molecular mechanisms of the preovulatory follicle differenciation and ovulation: What do we know in the mare relative to other species. Theriogenology 2019, 130, 163–176. [Google Scholar] [CrossRef]
Hinrichs, K. Assisted reproductive techniques in mares. Reprod. Dom. Anim. 2018, 53, 4–13. [Google Scholar] [CrossRef] [PubMed]
Child, T.J.; Abdul-Jalil, A.K.; Gulekli, B.; Lin Tan, S. In vitro maturation and fertilization of oocytes from unstimulated normal ovaries, polycystic ovaries, and women with polycystic ovary syndrome. Fertil. Steril. 2001, 76, 936–942. [Google Scholar] [CrossRef]
Le Du, A.; Kadoch, I.J.; Bourcigaux, N.; Doumerc, S.; Bourrier, M.-C.; Chevalier, N.; Fanchin, R.; Chian, R.-C.; Tachdjian, G.; Frydman, R.; et al. In vitro oocyte maturation for the treatment of infertility associated with polycystic ovarian syndrome: The French experience. Hum. Reprod. 2005, 20, 420–424. [Google Scholar] [CrossRef]
Grynberg, M.; Poulain, M.; le Parco, S.; Sifer, C.; Fanchin, R.; Frydman, N. Similar in vitro maturation rates of oocytes retrieved during the follicular or luteal phase offer flexible options for urgent fertility preservation in breast cancer patients. Hum. Reprod 2016, 31, 623–629. [Google Scholar] [CrossRef] [PubMed]
Hourvitz, A.; Maman, E.; Brengauz, M.; Machtinger, R.; Dor, J. In vitro maturation for patients with repeated in vitro fertilization failure due to “oocyte maturation abnormalities”. Fertil. Steril. 2010, 94, 496–501. [Google Scholar] [CrossRef]
Galvão, A.; Segers, I.; Smitz, J.; Tournaye, H.; De Vos, M. In vitro maturation (IVM) of oocytes in patients with resistant ovary syndrome and in patients with repeated deficient oocyte maturation. J. Assist. Reprod. Genet.2018, 35, 2161–2171. [Google Scholar] [CrossRef]
Liu, J.; Lu, G.; Qian, Y.; Mao, Y.; Ding, W. Pregnancies and births achieved from in vitro matured oocytes retrieved from poor responders undergoing stimulation in in vitro fertilization cycles. Fertil. Steril. 2003, 80, 447–449. [Google Scholar] [CrossRef]
Grynberg, M.; Peltoketo, H.; Christin-Maître, S.; Poulain, M.; Bouchard, P.; Fanchin, R. First birth achieved after in vitro maturation of oocytes from a woman endowed with multiple antral follicles unresponsive to follicle-stimulating hormone. J. Clin. Endocrinol. Metab. 2013, 98, 4493–4498. [Google Scholar] [CrossRef] [PubMed]
Benammar, A.; Fanchin, R.; Filali-Baba, M.; Vialard, F.; Fossard, C.; Vandame, J.; Pirtea, P.; Racowsky, C.; Ayoubi, J.-M.; Poulain, M. Utilization of in vitro maturation in cases with a FSH receptor mutation. J. Assist. Reprod. Genet. 2021. [Google Scholar] [CrossRef] [PubMed]
Vuong, L.N.; Ho, V.N.A.; Ho, T.M.; Dang, V.Q.; Phung, T.H.; Giang, N.H.; Le, A.H.; Pham, T.D.; Wang, R.; Smitz, J.; et al. In-vitro maturation of oocytes versus conventional IVF in women with infertility and a high antral follicle count: A randomized non-inferiority controlled trial. Hum. Reprod. 2020, 35, 2537–2547. [Google Scholar] [CrossRef]
Mackens, S.; Mostinckx, L.; Drakopoulos, P.; Segers, I.; Santos-Ribeiro, S.; Popovic-Todorovic, B.; Tournaye, H.; Blockeel, C.; De Vos, M. Early pregnancy loss in patients with polycystic ovary syndrome after IVM versus standard ovarian stimulation for IVF/ICSI. Hum. Reprod. 2020, 35, 2763–2773. [Google Scholar] [CrossRef]
Gliozheni, O.; Hambartsoumian, E.; Strohmer, H.; Petrovskaya, E.; Tishkevich, O.; Bogaerts, K.; Wyns, C.; Balic, D.; Antonova, I.; The European IVF-monitoring Consortium (EIM)‡ for the European Society of Human Reproduction and Embryology (ESHRE); et al. ART in Europe, 2016: Results generated from European registries by ESHRE. Hum. Reprod. Open 2020, 2020, hoaa032. [Google Scholar] [CrossRef]
Hinrichs, K. Advances in holding and cryopreservation of equine oocytes and embryos. J. Equine Vet. Sci.2020, 89, 102990. [Google Scholar] [CrossRef]
Riera, F.L.; Roldán, J.E.; Espinosa, J.M.; Fernandez, J.E.; Ortiz, I.; Hinrichs, K. Application of embryo biopsy and sex determination via polymerase chain reaction in a commercial equine embryo transfer program in Argentina. Reprod. Fertil. Dev. 2019, 31, 1917. [Google Scholar] [CrossRef]
Foss, R.; Ortis, H.; Hinrichs, K. Effect of potential oocyte transport protocols on blastocyst rates after intracytoplasmic sperm injection in the horse: Transport of equine oocytes for ICSI. Equine Vet. J. 2013, 45, 39–43. [Google Scholar] [CrossRef] [PubMed]
Pickering, S.J.; Johnson, M.H.; Braude, P.R.; Houliston, E. Cytoskeletal organization in fresh, aged and spontaneously activated human oocytes. Hum. Reprod. 1988, 3, 978–989. [Google Scholar] [CrossRef]
Wang, W.-H. Meiotic spindle, spindle checkpoint and embryonic aneuploidy. Front. Biosci. 2006, 11, 620. [Google Scholar] [CrossRef]
Chian, R.-C.; Buckett, W.M.; Tan, S.-L. In-vitro maturation of human oocytes. Reprod. Biomed. Online 2004, 8, 148–166. [Google Scholar] [CrossRef]
Lane, M.; Gardner, D.K. Regulation of ionic homeostasis by mammalian embryos. Semin. Reprod. Med. 2000, 18, 195–204. [Google Scholar] [CrossRef] [PubMed]
Castelbaum, A.J.; Freedman, M.F. Is there a role for gamete intra-fallopian transfer and other tubal insemination procedures? Curr. Opin. Obs. Gynecol. 1998, 10, 239–242. [Google Scholar] [CrossRef] [PubMed]
Squires, E.L.; Carnevale, E.M.; McCue, P.M.; Bruemmer, J.E. Embryo technologies in the horse. Theriogenology 2003, 59, 151–170. [Google Scholar] [CrossRef]
Squires, E. Current reproductive technologies impacting equine embryo production. J. Equine Vet. Sci. 2020, 89, 102981. [Google Scholar] [CrossRef]
Squires, E.L. Perspectives on the development and incorporation of assisted reproduction in the equine industry. Reprod. Fertil. Dev. 2019, 31, 1753. [Google Scholar] [CrossRef] [PubMed]
Palermo, G.; Joris, H.; Devroey, P.; Van Steirteghem, A.C. Pregnancies after intracytoplasmic injection of single spermatozoon into an oocyte. Lancet 1992, 340, 17–18. [Google Scholar] [CrossRef]
Esteves, S.C.; Miyaoka, R.; Agarwal, A. An update on the clinical assessment of the infertile male. Clinics 2011, 66, 691–700. [Google Scholar] [CrossRef]
Zegers-Hochschild, F.; Adamson, G.D.; Dyer, S.; Racowsky, C.; de Mouzon, J.; Sokol, R.; Rienzi, L.; Sunde, A.; Schmidt, L.; Cooke, I.D.; et al. The International glossary on infertility and fertility care, 2017†‡§. Hum. Reprod.2017, 32, 1786–1801. [Google Scholar] [CrossRef] [PubMed]
Esteves, S.C.; Roque, M.; Bedoschi, G.; Haahr, T.; Humaidan, P. Intracytoplasmic sperm injection for male infertility and consequences for offspring. Nat. Rev. Urol. 2018, 15, 535–562. [Google Scholar] [CrossRef]
Dyer, S.; Chambers, G.M.; de Mouzon, J.; Nygren, K.G.; Zegers-Hochschild, F.; Mansour, R.; Ishihara, O.; Banker, M.; Adamson, G.D. International committee for monitoring assisted reproductive technologies world report: Assisted reproductive technology 2008, 2009 and 2010†. Hum. Reprod. 2016, 31, 1588–1609. [Google Scholar] [CrossRef]
Grimstad, F.W.; Nangia, A.K.; Luke, B.; Stern, J.E.; Mak, W. Use of ICSI in IVF cycles in women with tubal ligation does not improve pregnancy or live birth rates. Hum. Reprod. 2016, 31, 1–6. [Google Scholar] [CrossRef]
Chambers, G.M.; Wand, H.; Macaldowie, A.; Chapman, M.G.; Farquhar, C.M.; Bowman, M.; Molloy, D.; Ledger, W. Population trends and live birth rates associated with common ART treatment strategies. Hum. Reprod.2016, 31, 2632–2641. [Google Scholar] [CrossRef]
The Practice Committees of the American Society for Reproductive Medicine and Society for Assisted Reproductive Technology. Intracytoplasmic sperm injection (ICSI) for non-male factor infertility: A committee opinion. Fertil. Steril. 2012, 98, 1395–1399. [Google Scholar] [CrossRef]
Palmer, E.; Bézard, J.; Magistrini, M.; Duchamp, G. In vitro fertilization in the horse. A Retrosp. Study J. Reprod. Fert. 1991, 44, 375–384. [Google Scholar]
Choi, Y.H.; Okada, Y.; Hochi, S.; Braun, J.; Sato, K.; Oguri, N. In vitro fertilization rate of horse oocytes with partially removed zonae. Theriogenology 1994, 42, 795–802. [Google Scholar] [CrossRef]
Smits, K.; Govaere, J.; Hoogewijs, M.; Piepers, S.; Van Soom, A. A pilot comparison of laser-assisted vs piezo drill icsi for the in vitro production of horse embryos: Icsi in horses: Laser Vs Piezo. Reprod. Domest. Anim.2012, 47, e1–e3. [Google Scholar] [CrossRef] [PubMed]
Oguri, N.; Tsutsumi, Y. Non-surgical egg transfer in mares. Reproduction 1974, 41, 313–320. [Google Scholar] [CrossRef]
Douglas, R.H. Review of induction of superovulation and embryo transfer in the equine. Theriogenology 1979, 11, 33–46. [Google Scholar] [CrossRef]
Duranthon, V.; Chavatte-Palmer, P. Long term effects of ART: What do animals tell us? Mol. Reprod. Dev. 2018, 85, 348–368. [Google Scholar] [CrossRef] [PubMed]
Mortimer, D.; Cohen, J.; Mortimer, S.T.; Fawzy, M.; McCulloh, D.H.; Morbeck, D.E.; Pollet-Villard, X.; Mansour, R.T.; Brison, D.R.; Doshi, A.; et al. Cairo consensus on the IVF laboratory environment and air quality: Report of an expert meeting. Reprod. Biomed. Online 2018, 36, 658–674. [Google Scholar] [CrossRef]
De los Santos, M.J.; Apter, S.; Coticchio, G.; Debrock, S.; Lundin, K.; Plancha, C.E.; Prados, F.; Rienzi, L.; Verheyen, G.; Eshre Guideline Group on Good Practice in IVF Labs; et al. Revised Guidelines for good practice in IVF laboratories (2015)†. Hum. Reprod. 2016, 31, 685–686. [Google Scholar] [CrossRef]
Swain, J. Optimal human embryo culture. Semin. Reprod. Med 2015, 33, 103–117. [Google Scholar] [CrossRef]
Swain, J.E.; Carrell, D.; Cobo, A.; Meseguer, M.; Rubio, C.; Smith, G.D. Optimizing the culture environment and embryo manipulation to help maintain embryo developmental potential. Fertil. Steril. 2016, 105, 571–587. [Google Scholar] [CrossRef]
Hinrichs, K. In vitro production of equine embryos: State of the art: In vitro production of equine embryos. Reprod. Domest. Anim. 2010, 45, 3–8. [Google Scholar] [CrossRef] [PubMed]
The Vienna consensus: Report of an expert meeting on the development of ART laboratory performance indicators. Reprod. Biomed. Online 2017, 35, 494–510. [CrossRef] [PubMed]
Carnevale, E.M.; Metcalf, E.S. Morphology, developmental stages and quality parameters of in vitro-produced equine embryos. Reprod. Fertil. Dev. 2019, 31, 1758. [Google Scholar] [CrossRef] [PubMed]
Tremoleda, J.L.; Stout, T.A.E.; Lagutina, I.; Lazzari, G.; Bevers, M.M.; Colenbrander, B.; Galli, C. Effects of in vitro production on horse embryo morphology, cytoskeletal characteristics, and blastocyst capsule formation. Biol. Reprod. 2003, 69, 1895–1906. [Google Scholar] [CrossRef] [PubMed]
Choi, Y.H.; Harding, H.D.; Hartman, D.L.; Obermiller, A.D.; Kurosaka, S.; McLaughlin, K.J.; Hinrichs, K. The uterine environment modulates trophectodermal POU5F1 levels in equine blastocysts. Reproduction 2009, 138, 589–599. [Google Scholar] [CrossRef]
Simopoulou, M.; Sfakianoudis, K.; Maziotis, E.; Tsioulou, P.; Grigoriadis, S.; Rapani, A.; Giannelou, P.; Asimakopoulou, M.; Kokkali, G.; Pantou, A.; et al. PGT-A: Who and when? A systematic review and network meta-analysis of RCTs. J. Assist. Reprod. Genet. 2021. [Google Scholar] [CrossRef]
Troedsson, M.H.T.; Paprocki, A.M.; Koppang, R.W.; Syverson, C.M.; Griffin, P.G.; Klein, C.; Dobrinsky, J.R. Transfer success of biopsied and vitrified equine embryos. Anim. Reprod. Sci. 2010, 121, 295–296. [Google Scholar] [CrossRef]
Sciorio, R. Use of time-lapse monitoring in medically assisted reproduction treatments: A mini-review. Zygote2021, 29, 93–101. [Google Scholar] [CrossRef]
Brooks, K.E.; Daughtry, B.L.; Metcalf, E.; Masterson, K.; Battaglia, D.; Gao, L.; Park, B.; Chavez, S.L. Assessing equine embryo developmental competency by time-lapse image analysis. Reprod. Fertil. Dev. 2019, 31, 1840. [Google Scholar] [CrossRef]
Lewis, N.; Schnauffer, K.; Hinrichs, K.; Morganti, M.; Troup, S.; Argo, C. Morphokinetics of early equine embryo development in vitro using time-lapse imaging, and use in selecting blastocysts for transfer. Reprod. Fertil. Dev.2019, 31, 1851. [Google Scholar] [CrossRef]
Martino, N.A.; Marzano, G.; Mastrorocco, A.; Lacalandra, G.M.; Vincenti, L.; Hinrichs, K.; Dell’Aquila, M.E. Use of time-lapse imaging to evaluate morphokinetics of in vitro equine blastocyst development after oocyte holding for two days at 15°C versus room temperature before intracytoplasmic sperm injection. Reprod. Fertil. Dev.2019, 31, 1862. [Google Scholar] [CrossRef] [PubMed]
Meyers, S.; Burruel, V.; Kato, M.; de la Fuente, A.; Orellana, D.; Renaudin, C.; Dujovne, G. Equine non-invasive time-lapse imaging and blastocyst development. Reprod. Fertil. Dev. 2019, 31, 1874. [Google Scholar] [CrossRef] [PubMed]
Hernández-Vargas, P.; Muñoz, M.; Domínguez, F. Identifying biomarkers for predicting successful embryo implantation: Applying single to multi-OMICs to improve reproductive outcomes. Hum. Reprod. Update 2020, 26, 264–301. [Google Scholar] [CrossRef]
Handyside, A.H.; Kontogianni, E.H.; Hardy, K.; Winston, R.M.L. Pregnancies from biopsied human preimplantation embryos sexed by Y-specific DNA amplification. Nature 1990, 344, 768–770. [Google Scholar] [CrossRef]
Verlinsky, Y.; Ginsberg, N.; Lifchez, A.; Valle, J.; Moise, J.; Strom, C.M. Analysis of the first polar body: Preconception genetic diagnosis. Hum. Reprod. 1990, 5, 826–829. [Google Scholar] [CrossRef]
Hinrichs, K.; Choi, Y.-H. Equine embryo biopsy, genetic testing, and cryopreservation. J. Equine Vet. Sci. 2012, 32, 390–396. [Google Scholar] [CrossRef]
Herrera, C.; Morikawa, M.I.; Bello, M.B.; von Meyeren, M.; Eusebio Centeno, J.; Dufourq, P.; Martinez, M.M.; Llorente, J. Setting up equine embryo gender determination by preimplantation genetic diagnosis in a commercial embryo transfer program. Theriogenology 2014, 81, 758–763. [Google Scholar] [CrossRef]
Choi, Y.H.; Penedo, M.C.T.; Daftari, P.; Velez, I.C.; Hinrichs, K. Accuracy of preimplantation genetic diagnosis in equine in vivo-recovered and in vitro-produced blastocysts. Reprod. Fertil. Dev. 2016, 28, 1382. [Google Scholar] [CrossRef]
Barandalla, M.; Benedetti, M.; Colleoni, S.; Perota, A.; Galli, C.; Lazzari, G. Genetic diagnosis of Warmblood Fragile Foal Syndrome in equine in vitro produced preimplantation embryos. J. Equine Vet. Sci. 2020, 89, 103047. [Google Scholar] [CrossRef]
Cimadomo, D.; Rienzi, L.; Capalbo, A.; Rubio, C.; Innocenti, F.; García-Pascual, C.M.; Ubaldi, F.M.; Handyside, A. The dawn of the future: 30 years from the first biopsy of a human embryo. Detail. Hist. Ongoing Revolut. Hum. Reprod. Update 2020, 26, 453–473. [Google Scholar] [CrossRef]
Leaver, M.; Wells, D. Non-invasive preimplantation genetic testing (niPGT): The next revolution in reproductive genetics? Hum. Reprod. Update 2020, 26, 16–42. [Google Scholar] [CrossRef]
Herrera, C.; Morikawa, M.I.; Castex, C.B.; Pinto, M.R.; Ortega, N.; Fanti, T.; Garaguso, R.; Franco, M.J.; Castañares, M.; Castañeira, C.; et al. Blastocele fluid from in vitro– and in vivo–produced equine embryos contains nuclear DNA. Theriogenology 2015, 83, 415–420. [Google Scholar] [CrossRef] [PubMed]
Leibo, S.P.; Sztein, J.M. Cryopreservation of mammalian embryos: Derivation of a method. Cryobiology 2019, 86, 1–9. [Google Scholar] [CrossRef] [PubMed]
Trounson, A.; Mohr, L. Human pregnancy following cryopreservation, thawing and transfer of an eight-cell embryo. Nature 1983, 305, 707–709. [Google Scholar] [CrossRef] [PubMed]
Downing, B.G.; Mohr, L.R.; Trounson, A.O.; Freeman, L.E.; Wood, C. Birth after transfer of cryopreserved embryos. Med. J. Aust. 1985, 142, 409–411. [Google Scholar] [CrossRef]
Yamamoto, Y.; Oguri, N.; Tsutsumi, Y.; Hachinohe, Y. Experiments in the freezing and storage of equine embryos. J. Reprod. Fertil. Suppl. 1982, 32, 399–403. [Google Scholar]
Edgar, D.H.; Gook, D.A. A critical appraisal of cryopreservation (slow cooling versus vitrification) of human oocytes and embryos. Hum. Reprod. Update 2012, 18, 536–554. [Google Scholar] [CrossRef] [PubMed]
Squires, E.L.; McCue, P.M. Cryopreservation of equine embryos. J. Equine Vet. Sci. 2016, 41, 7–12. [Google Scholar] [CrossRef]
Carnevale, E.M.; Squires, E.L. Comparison of ham’s F 1 0 W I T H CO2 or hepes buffer for storage of equine embryos at 5 C for 24 H l. J. Anim. Sci. 1987, 65, 1775–1781. [Google Scholar] [CrossRef] [PubMed]
Rienzi, L.; Gracia, C.; Maggiulli, R.; LaBarbera, A.R.; Kaser, D.J.; Ubaldi, F.M.; Vanderpoel, S.; Racowsky, C. Oocyte, embryo and blastocyst cryopreservation in ART: Systematic review and meta-analysis comparing slow-freezing versus vitrification to produce evidence for the development of global guidance. Hum. Reprod. Update2017, 23, 139–155. [Google Scholar] [CrossRef]
Zaat, T.; Zagers, M.; Mol, F.; Goddijn, M.; van Wely, M.; Mastenbroek, S. Fresh versus frozen embryo transfers in assisted reproduction. Cochrane Database Syst. Rev. 2021, 2, CD011184. [Google Scholar] [CrossRef]
Galli, C.; Colleoni, S.; Duchi, R.; Lagutina, I.; Lazzari, G. Developmental competence of equine oocytes and embryos obtained by in vitro procedures ranging from in vitro maturation and ICSI to embryo culture, cryopreservation and somatic cell nuclear transfer. Anim. Reprod. Sci. 2007, 98, 39–55. [Google Scholar] [CrossRef]
Choi, Y.-H.; Hinrichs, K. Vitrification of in vitro -produced and in vivo -recovered equine blastocysts in a clinical program. Theriogenology 2017, 87, 48–54. [Google Scholar] [CrossRef] [PubMed]
Van Landuyt, L.; Polyzos, N.P.; De Munck, N.; Blockeel, C.; Van de Velde, H.; Verheyen, G. A prospective randomized controlled trial investigating the effect of artificial shrinkage (collapse) on the implantation potential of vitrified blastocysts. Hum. Reprod. 2015, 30, 2509–2518. [Google Scholar] [CrossRef]
Choi, Y.H.; Velez, I.C.; Riera, F.L.; Roldán, J.E.; Hartman, D.L.; Bliss, S.B.; Blanchard, T.L.; Hayden, S.S.; Hinrichs, K. Successful cryopreservation of expanded equine blastocysts. Theriogenology 2011, 76, 143–152. [Google Scholar] [CrossRef]
Wilsher, S.; Rigali, F.; Kovacsy, S.; Allen, W.R. Successful vitrification of manually punctured equine embryos. Equine Vet. J. 2021, evj.13400. [Google Scholar] [CrossRef]
Chen, S.-U.; Lien, Y.-R.; Chang, L.-J.; Tsai, Y.-Y.; Ho, H.-N.; Yang, Y.-S. Short communication: Cryopreserved sibling oocytes and intracytoplasmic sperm injection rescue unexpectedly poor fertilization in conventional in vitro fertilization. J. Assist. Reprod. Genet. 2004, 21, 367–369. [Google Scholar] [CrossRef] [PubMed]
Roberts, J.E. Fertility preservation: A Comprehensive approach to the young woman with cancer. J. Natl. Cancer Inst. Monogr. 2005, 2005, 57–59. [Google Scholar] [CrossRef] [PubMed]
Clark, N.A.; Swain, J.E. Oocyte cryopreservation: Searching for novel improvement strategies. J. Assist. Reprod. Genet. 2013, 30, 865–875. [Google Scholar] [CrossRef]
Maclellan, L.J.; Carnevale, E.M.; Scoggin, C.F.; Bruemmer, J.E.; Squires, E.L. Pregnancies from vitrified equine oocytes collected from super-stimulated and non-stimulated mares. Theriogenology 2002, 58, 911–919. [Google Scholar] [CrossRef]
Ortiz-Escribano, N.; Bogado Pascottini, O.; Woelders, H.; Vandenberghe, L.; De Schauwer, C.; Govaere, J.; Van den Abbeel, E.; Vullers, T.; Ververs, C.; Roels, K.; et al. An improved vitrification protocol for equine immature oocytes, resulting in a first live foal. Equine Vet. J. 2018, 50, 391–397. [Google Scholar] [CrossRef] [PubMed]
Canesin, H.S.; Brom-de-Luna, J.G.; Choi, Y.-H.; Ortiz, I.; Diaw, M.; Hinrichs, K. Blastocyst development after intracytoplasmic sperm injection of equine oocytes vitrified at the germinal-vesicle stage. Cryobiology 2017, 75, 52–59. [Google Scholar] [CrossRef]
Ducheyne, K.D.; Rizzo, M.; Daels, P.F.; Stout, T.A.E.; De Ruijter-Villani, M. Vitrifying immature equine oocytes impairs their ability to correctly align the chromosomes on the MII spindle. Reprod. Fertil. Dev. 2019, 31, 1330. [Google Scholar] [CrossRef] [PubMed]
Angel, D.; Canesin, H.S.; Brom-de-Luna, J.G.; Morado, S.; Dalvit, G.; Gomez, D.; Posada, N.; Pascottini, O.B.; Urrego, R.; Hinrichs, K.; et al. Embryo development after vitrification of immature and in vitro-matured equine oocytes. Cryobiology 2020, 92, 251–254. [Google Scholar] [CrossRef]
Jeffcott, L.B.; Rossdale, P.D.; Freestone, J.; Frank, C.J.; Towers-Clark, P.F. An assessment of wastage in Thoroughbred racing from conception to 4 years of age. Equine Vet. J. 1982, 14, 185–198. [Google Scholar] [CrossRef]
Langlois, B.; Blouin, C. Statistical analysis of some factors affecting the number of horse births in France. Reprod. Nutr. Dev. 2004, 44, 583–595. [Google Scholar] [CrossRef]
Cuervo-Arango, J.; Claes, A.N.; Stout, T.A. A retrospective comparison of the efficiency of different assisted reproductive techniques in the horse, emphasizing the impact of maternal age. Theriogenology 2019, 132, 36–44. [Google Scholar] [CrossRef] [PubMed]
Carnevale, E.M.; Ginther, O.J. Relationships of age to uterine function and reproductive efficiency in mares. Theriogenology 1992, 37, 1101–1115. [Google Scholar] [CrossRef]
Carnevale, E.M.; Bergfelt, D.R.; Ginther, O.J. Aging effects on follicular activity and concentrations of FSH, LH, and progesterone in mares. Anim. Reprod. Sci. 1993, 31, 287–299. [Google Scholar] [CrossRef]
Rizzo, M.; Ducheyne, K.D.; Deelen, C.; Beitsma, M.; Cristarella, S.; Quartuccio, M.; Stout, T.A.E.; Ruijter-Villani, M. Advanced mare age impairs the ability of in vitro-matured oocytes to correctly align chromosomes on the metaphase plate. Equine Vet. J. 2019, 51, 252–257. [Google Scholar] [CrossRef] [PubMed]
Campos-Chillon, F.; Farmerie, T.A.; Bouma, G.J.; Clay, C.M.; Carnevale, E.M. Effects of aging on gene expression and mitochondrial DNA in the equine oocyte and follicle cells. Reprod. Fertil. Dev. 2015, 27, 925. [Google Scholar] [CrossRef]
Rambags, B.P.B.; Van Boxtel, D.C.J.; Tharasanit, T.; Lenstra, J.A.; Colenbrander, B.; Stout, T.A.E. Advancing maternal age predisposes to mitochondrial damage and loss during maturation of equine oocytes in vitro. Theriogenology 2014, 81, 959–965. [Google Scholar] [CrossRef]
Cox, L.; Vanderwall, D.K.; Parkinson, K.C.; Sweat, A.; Isom, S.C. Expression profiles of select genes in cumulus–oocyte complexes from young and aged mares. Reprod. Fertil. Dev. 2015, 27, 914. [Google Scholar] [CrossRef] [PubMed]
Doig, P.A.; Mcknight, J.D.; Miller, R.B. The use of endometrial biopsy in the infertile mare. Can. Vet. J. 1981, 22, 72–76. [Google Scholar]
Siemieniuch, M.J.; Gajos, K.; Kozdrowski, R.; Nowak, M. Advanced age in mares affects endometrial secretion of arachidonic acid metabolites during equine subclinical endometritis. Theriogenology 2017, 103, 191–196. [Google Scholar] [CrossRef] [PubMed]
Nambo, Y.; Oikawa, M.-A.; Yoshihara, T.; Kuwano, A.; Katayama, Y. Age-related morphometrical changes of arteries of uterine wall in mares. J. Vet. Med. Ser. A 1995, 42, 383–387. [Google Scholar] [CrossRef]
Oikawa, M.; Katayama, Y.; Yoshihara, T.; Kaneko, M.; Yoshikawa, T. Microscopical characteristics of uterine wall arteries in barren aged mares. J. Comp. Pathol. 1993, 108, 411–415. [Google Scholar] [CrossRef]
Dubrovskaya, A.B.; Lebedeva, L.F.; Schukis, K.A. Comparative histomorphological characteristics of the endometrium of young and aged mares in estrus and diestrus. IOP Conf. Ser. Earth Environ. Sci. 2019, 341, 012067. [Google Scholar] [CrossRef]
Pellestor, F.; Andréo, B.; Arnal, F.; Humeau, C.; Demaille, J. Maternal aging and chromosomal abnormalities: New data drawn from in vitro unfertilized human oocytes. Hum. Genet. 2003, 112, 195–203. [Google Scholar] [CrossRef]
Hassold, T.; Hunt, P. To err (meiotically) is human: The genesis of human aneuploidy. Nat. Rev. Genet. 2001, 2, 280–291. [Google Scholar] [CrossRef]
Ball, B.A.; Little, T.V.; Hillman, R.B.; Woods, G.L. Pregnancy rates at Days 2 and 14 and estimated embryonic loss rates prior to day 14 in normal and subfertile mares. Theriogenology 1986, 26, 611–619. [Google Scholar] [CrossRef]
Carnevale, E.M.; Ginther, O.J. Defective oocytes as a cause of subfertility in old mares. Biol. Reprod. 1995, 52, 209–214. [Google Scholar] [CrossRef]
Ruggeri, E.; DeLuca, K.F.; Galli, C.; Lazzari, G.; DeLuca, J.G.; Carnevale, E.M. Cytoskeletal alterations associated with donor age and culture interval for equine oocytes and potential zygotes that failed to cleave after intracytoplasmic sperm injection. Reprod. Fertil. Dev. 2015, 27, 944. [Google Scholar] [CrossRef] [PubMed]
Brinsko, S.P.; Ball, B.A.; Ellington, J.E. In vitro maturation of equine oocytes obtained from different age groups of sexually mature mares. Theriogenology 1995, 44, 461–469. [Google Scholar] [CrossRef]
Rizzo, M.; Deelen, C.; Beitsma, M.; Stout, T.A.; De Ruijter-Villani, M. In vitro matured horse oocytes from aged mares show weakened centromeric cohesion and a higher incidence of aneuploidy. J. Equine Vet. Sci. 2020, 89, 103028. [Google Scholar] [CrossRef]
Shilton, C.A.; Kahler, A.; Davis, B.W.; Crabtree, J.R.; Crowhurst, J.; McGladdery, A.J.; Wathes, D.C.; Raudsepp, T.; de Mestre, A.M. Whole genome analysis reveals aneuploidies in early pregnancy loss in the horse. Sci. Rep.2020, 10, 13314. [Google Scholar] [CrossRef]
Vialard, F.; Petit, C.; Bergere, M.; Gomes, D.M.; Martel-Petit, V.; Lombroso, R.; Ville, Y.; Gerard, H.; Selva, J. Evidence of a high proportion of premature unbalanced separation of sister chromatids in the first polar bodies of women of advanced age. Hum. Reprod. 2006, 21, 1172–1178. [Google Scholar] [CrossRef]
Rizzo, M.; Stout, T.A.E.; Cristarella, S.; Quartuccio, M.; Kops, G.J.P.L.; De Ruijter-Villani, M. Compromised MPS1 activity induces multipolar spindle formation in oocytes from aged mares: Establishing the horse as a natural animal model to study age-induced oocyte meiotic spindle instability. Front. Cell Dev. Biol. 2021, 9, 657366. [Google Scholar] [CrossRef]
Smits, M.A.J.; Wong, K.M.; Mantikou, E.; Korver, C.M.; Jongejan, A.; Breit, T.M.; Goddijn, M.; Mastenbroek, S.; Repping, S. Age-related gene expression profiles of immature human oocytes. Mol. Hum. Reprod. 2018, 24, 469–477. [Google Scholar] [CrossRef] [PubMed]
Morimoto, N.; Hashimoto, S.; Yamanaka, M.; Nakano, T.; Satoh, M.; Nakaoka, Y.; Iwata, H.; Fukui, A.; Morimoto, Y.; Shibahara, H. Mitochondrial oxygen consumption rate of human embryos declines with maternal age. J. Assist. Reprod. Genet. 2020, 37, 1815–1821. [Google Scholar] [CrossRef] [PubMed]
Jiang, Z.; Shen, H. Mitochondria: Emerging therapeutic strategies for oocyte rescue. Reprod. Sci. 2021. [Google Scholar] [CrossRef] [PubMed]
Grøndahl, M.L.; Andersen, C.Y.; Bogstad, J.; Nielsen, F.C.; Meinertz, H.; Borup, R. Gene expression profiles of single human mature oocytes in relation to age. Hum. Reprod. 2010, 25, 957–968. [Google Scholar] [CrossRef]
Llonch, S.; Barragán, M.; Nieto, P.; Mallol, A.; Elosua-Bayes, M.; Lorden, P.; Ruiz, S.; Zambelli, F.; Heyn, H.; Vassena, R.; et al. Single human oocyte transcriptome analysis reveals distinct maturation stage-dependent pathways impacted by age. Aging Cell 2021, 20, e13360. [Google Scholar] [CrossRef] [PubMed]
Spacek, S.G.; Carnevale, E.M. Impact of equine and bovine oocyte maturation in follicular fluid from young and old mares on embryo production in vitro. J. Equine Vet. Sci. 2018, 68, 94–100. [Google Scholar] [CrossRef]
Hendriks, W.K.; Colleoni, S.; Galli, C.; Paris, D.B.B.P.; Colenbrander, B.; Roelen, B.A.J.; Stout, T.A.E. Maternal age and in vitro culture affect mitochondrial number and function in equine oocytes and embryos. Reprod. Fertil. Dev. 2015, 27, 957. [Google Scholar] [CrossRef]
Derisoud, E.; Jouneau, L.; Dubois, C.; Archilla, C.; Jaszczyszyn, Y.; Legendre, R.; Daniel, N.; Peynot, N.; Dahirel, M.; Auclair-Ronzaud, J.; et al. Maternal age affects equine Day 8 embryo gene expression both in trophoblast and inner cell mass. BioRxiv 2021, 438786. [Google Scholar] [CrossRef]
Coi, A.; Santoro, M.; Garne, E.; Pierini, A.; Addor, M.-C.; Alessandri, J.-L.; Bergman, J.E.H.; Bianchi, F.; Boban, L.; Braz, P.; et al. Epidemiology of achondroplasia: A population-based study in Europe. Am. J. Med. Genet. A2019, 179, 1791–1798. [Google Scholar] [CrossRef] [PubMed]
Sandin, S.; Schendel, D.; Magnusson, P.; Hultman, C.; Surén, P.; Susser, E.; Grønborg, T.; Gissler, M.; Gunnes, N.; Gross, R.; et al. Autism risk associated with parental age and with increasing difference in age between the parents. Mol. Psychiatry 2016, 21, 693–700. [Google Scholar] [CrossRef] [PubMed]
Shinde, D.N.; Elmer, D.P.; Calabrese, P.; Boulanger, J.; Arnheim, N.; Tiemann-Boege, I. New evidence for positive selection helps explain the paternal age effect observed in achondroplasia. Hum. Mol. Genet. 2013, 22, 4117–4126. [Google Scholar] [CrossRef]
World Health Organization. Obesity and overweight. Fact Sheets. 2021. Available online: https://www.who.int/news-room/fact-sheets/detail/obesity-and-overweight (accessed on 22 June 2021).
Gallus, S.; Lugo, A.; Murisic, B.; Bosetti, C.; Boffetta, P.; La Vecchia, C. Overweight and obesity in 16 European countries. Eur. J. Nutr. 2015, 54, 679–689. [Google Scholar] [CrossRef]
Jensen, R.B.; Danielsen, S.H.; Tauson, A.-H. Body condition score, morphometric measurements and estimation of body weight in mature Icelandic horses in Denmark. Acta. Vet. Scand. 2016, 58, 59. [Google Scholar] [CrossRef]
Wyse, C.A.; McNie, K.A.; Tannahil, V.J.; Murray, J.K.; Love, S. Prevalence of obesity in riding horses in Scotland. Vet. Rec. 2008, 162, 590–591. [Google Scholar] [CrossRef] [PubMed]
Thatcher, C.D.; Pleasant, R.S.; Geor, R.J.; Elvinger, F. Prevalence of Overconditioning in Mature Horses in Southwest Virginia during the Summer. J. Vet. Intern. Med. 2012, 26, 1413–1418. [Google Scholar] [CrossRef] [PubMed]
Harker, I.J.; Harris, P.A.; Barfoot, C.F. The body condition score of leisure horses competing at an unaffiliated championship in the UK. J. Equine Vet. Sci. 2011, 31, 253–254. [Google Scholar] [CrossRef]
Frank, N.; Geor, R.J.; Bailey, S.R.; Durham, A.E.; Johnson, P.J. American college of veterinary internal, m. equine metabolic syndrome. J. Vet. Intern. Med./Am. Coll. Vet. Intern. Med. 2010, 24, 467–475. [Google Scholar] [CrossRef] [PubMed]
Engin, A. The definition and prevalence of obesity and metabolic syndrome. In Obesity and Lipotoxicity; Engin, A.B., Engin, A., Eds.; Springer International Publishing: Cham, Switzerland, 2017; Volume 960, pp. 1–17. [Google Scholar] [CrossRef]
Grieger, J.A.; Hutchesson, M.J.; Cooray, S.D.; Bahri Khomami, M.; Zaman, S.; Segan, L.; Teede, H.; Moran, L.J. A review of maternal overweight and obesity and its impact on cardiometabolic outcomes during pregnancy and postpartum. Ther. Adv. Reprod. Health 2021, 15, 2633494120986544. [Google Scholar] [CrossRef] [PubMed]
Gesink Law, D.C.; Maclehose, R.F.; Longnecker, M.P. Obesity and time to pregnancy. Hum. Reprod. 2006, 22, 414–420. [Google Scholar] [CrossRef]
Incedal Irgat, S.; Bakirhan, H. The effect of obesity on human reproductive health and foetal life. Hum. Fertil.2021, 1–12. [Google Scholar] [CrossRef]
Sessions, D.R.; Reedy, S.E.; Vick, M.M.; Murphy, B.A.; Fitzgerald, B.P. Development of a model for inducing transient insulin resistance in the mare: Preliminary implications regarding the estrous cycle12. J. Anim. Sci.2004, 82, 2321–2328. [Google Scholar] [CrossRef]
Gentry, L.R.; Thompson, D.L.J.; Gentry, G.T.J.; Davis, K.A.; Godke, R.A.; Cartmill, J.A. The relationship between body condition, leptin, and reproductive and hormonal characteristics of mares during the seasonal anovulatory period. J. Anim. Sci. 2002, 80, 2695–2703. [Google Scholar] [CrossRef] [PubMed]
D’Fonseca, N.M.M.; Gibson, C.M.E.; Hummel, I.; van Doorn, D.A.; Roelfsema, E.; Stout, T.A.E.; van den Broek, J.; de Ruijter-Villani, M. Overfeeding extends the period of annual cyclicity but increases the risk of early embryonic death in shetland pony mares. Animals 2021, 11, 361. [Google Scholar] [CrossRef]
Waller, C.A.; Thompson, D.L.; Cartmill, J.A.; Storer, W.A.; Huff, N.K. Reproduction in high body condition mares with high versus low leptin concentrations. Theriogenology 2006, 66, 923–928. [Google Scholar] [CrossRef]
Provost, M.P.; Acharya, K.S.; Acharya, C.R.; Yeh, J.S.; Steward, R.G.; Eaton, J.L.; Goldfarb, J.M.; Muasher, S.J. Pregnancy outcomes decline with increasing body mass index: Analysis of 239,127 fresh autologous in vitro fertilization cycles from the 2008–2010 society for assisted reproductive technology registry. Fertil. Steril. 2016, 105, 663–669. [Google Scholar] [CrossRef]
Sermondade, N.; Huberlant, S.; Bourhis-Lefebvre, V.; Arbo, E.; Gallot, V.; Colombani, M.; Fréour, T. Female obesity is negatively associated with live birth rate following IVF: A systematic review and meta-analysis. Hum. Reprod. Update 2019, 25, 439–451. [Google Scholar] [CrossRef] [PubMed]
Si, C.; Wang, N.; Wang, M.; Liu, Y.; Niu, Z.; Ding, Z. TMT-based proteomic and bioinformatic analyses of human granulosa cells from obese and normal-weight female subjects. Reprod. Biol. Endocrinol. 2021, 19, 75. [Google Scholar] [CrossRef] [PubMed]
Sessions-Bresnahan, D.R.; Heuberger, A.L.; Carnevale, E.M. Obesity in mares promotes uterine inflammation and alters embryo lipid fingerprints and homeostasis†. Biol. Reprod. 2018, 99, 761–772. [Google Scholar] [CrossRef]
Vick, M.M.; Adams, A.A.; Murphy, B.A.; Sessions, D.R.; Horohov, D.W.; Cook, R.F.; Shelton, B.J.; Fitzgerald, B.P. Relationships among inflammatory cytokines, obesity, and insulin sensitivity in the horse1,2. J. Anim. Sci.2007, 85, 1144–1155. [Google Scholar] [CrossRef] [PubMed]
Pennington, P.M.; Splan, R.K.; Jacobs, R.D.; Chen, Y.; Singh, R.P.; Li, Y.; Gucek, M.; Wagner, A.L.; Freeman, E.W.; Pukazhenthi, B.S. Influence of Metabolic Status and Diet on Early Pregnant Equine Histotroph Proteome: Preliminary Findings. J. Equine Vet. Sci. 2020, 88, 102938. [Google Scholar] [CrossRef]
Sessions-Bresnahan, D.R.; Carnevale, E.M. The effect of equine metabolic syndrome on the ovarian follicular environment1. J. Anim. Sci. 2014, 92, 1485–1494. [Google Scholar] [CrossRef] [PubMed]
Sessions-Bresnahan, D.R.; Schauer, K.L.; Heuberger, A.L.; Carnevale, E.M. Effect of obesity on the preovulatory follicle and lipid fingerprint of equine oocytes1. Biol. Reprod. 2016, 94. [Google Scholar] [CrossRef]
Boutari, C.; Pappas, P.D.; Mintziori, G.; Nigdelis, M.P.; Athanasiadis, L.; Goulis, D.G.; Mantzoros, C.S. The effect of underweight on female and male reproduction. Metabolism 2020, 107, 154229. [Google Scholar] [CrossRef] [PubMed]
Kishali, N.F.; Imamoglu, O.; Katkat, D.; Atan, T.; Akyol, P. Effects of menstrual cycle on sports performance. Int. J. Neurosci. 2006, 116, 1549–1563. [Google Scholar] [CrossRef]
McNulty, K.L.; Elliott-Sale, K.J.; Dolan, E.; Swinton, P.A.; Ansdell, P.; Goodall, S.; Thomas, K.; Hicks, K.M. The effects of menstrual cycle phase on exercise performance in eumenorrheic women: A systematic review and meta-analysis. Sports Med. 2020, 50, 1813–1827. [Google Scholar] [CrossRef]
Heather, A.K.; Thorpe, H.; Ogilvie, M.; Sims, S.T.; Beable, S.; Milsom, S.; Schofield, K.L.; Coleman, L.; Hamilton, B. Biological and socio-cultural factors have the potential to influence the health and performance of elite female athletes: A cross sectional survey of 219 elite female athletes in aotearoa new zealand. Front. Sports Act. Living 2021, 3, 601420. [Google Scholar] [CrossRef]
Sundgot-Borgen, J.; Sundgot-Borgen, C.; Myklebust, G.; Sølvberg, N.; Torstveit, M.K. Elite athletes get pregnant, have healthy babies and return to sport early postpartum. BMJ Open Sport Exerc. Med. 2019, 5, e000652. [Google Scholar] [CrossRef]
Mortensen, C.J.; Choi, Y.H.; Hinrichs, K.; Ing, N.H.; Kraemer, D.C.; Vogelsang, S.G.; Vogelsang, M.M. Embryo recovery from exercised mares. Anim. Reprod. Sci. 2009, 110, 237–244. [Google Scholar] [CrossRef]
Kelley, D.E.; Gibbons, J.R.; Smith, R.; Vernon, K.L.; Pratt-Phillip, S.E.; Mortensen, C.J. Exercise affects both ovarian follicular dynamics and hormone concentrations in mares. Theriogenology 2011, 76, 615–622. [Google Scholar] [CrossRef] [PubMed]
Smith, R.L.; Vernon, K.L.; Kelley, D.E.; Gibbons, J.R.; Mortensen, C.J. Impact of moderate exercise on ovarian blood flow and early embryonic outcomes in mares1. J. Anim. Sci. 2012, 90, 3770–3777. [Google Scholar] [CrossRef]
Pessoa, M.A.; Cannizza, A.P.; Reghini, M.F.S.; Alvarenga, M.A. Embryo Transfer efficiency of quarter horse athletic mares. J. Equine Vet. Sci. 2011, 31, 703–705. [Google Scholar] [CrossRef]
Pinto, M.R.; Miragaya, M.H.; Burns, P.; Douglas, R.; Neild, D.M. Strategies for increasing reproductive efficiency in a commercial embryo transfer program with high performance donor mares under training. J. Equine Vet. Sci.2017, 54, 93–97. [Google Scholar] [CrossRef]
Sairanen, J.; Katila, T.; Virtala, A.-M.; Ojala, M. Effects of racing on equine fertility. Anim. Reprod. Sci. 2011, 124, 73–84. [Google Scholar] [CrossRef] [PubMed]
Anton, J.E.; Vernon, K.L.; Kelley, D.E.; Gibbons, J.R.; Birrenkott, G.; Mortensen, C.J. Exercising the pregnant mare from day 16 to day 80 of gestation. J. Equine Vet. Sci. 2014, 34, 415–420. [Google Scholar] [CrossRef]
Kilgenstein, H.J.; Schöniger, S.; Schoon, D.; Schoon, H.-A. Microscopic examination of endometrial biopsies of retired sports mares: An explanation for the clinically observed subfertility? Res. Vet. Sci. 2015, 99, 171–179. [Google Scholar] [CrossRef]
Farin, C.E.; Farmer, W.T.; Farin, P.W. Pregnancy recognition and abnormal offspring syndrome in cattle. Reprod. Fertil. Dev. 2010, 22, 75. [Google Scholar] [CrossRef]
Fleming, T.P.; Watkins, A.J.; Velazquez, M.A.; Mathers, J.C.; Prentice, A.M.; Stephenson, J.; Barker, M.; Saffery, R.; Yajnik, C.S.; Eckert, J.J.; et al. Origins of lifetime health around the time of conception: Causes and consequences. Lancet 2018, 391, 1842–1852. [Google Scholar] [CrossRef]
Kalbfleisch, T.S.; Rice, E.S.; DePriest, M.S.; Walenz, B.P.; Hestand, M.S.; Vermeesch, J.R.; O′Connell, B.L.; Fiddes, I.T.; Vershinina, A.O.; Saremi, N.F.; et al. Improved reference genome for the domestic horse increases assembly contiguity and composition. Commun. Biol. 2018, 1, 197. [Google Scholar] [CrossRef]
Okólski, A.; Bézard, J.; Duchamp, G.; Driancourt, M.-A.; Goudet, G.; Palmer, E. Successive Puncture of the dominant follicle followed by ovulation and fertilization: A new experimental model for the study of follicular maturation in the mare1. Biol. Reprod. 1995, 52, 385–392. [Google Scholar] [CrossRef]
Palmer, E.; Duchamp, G.; Cribiu, E.P.; Mahla, R.; Boyazoglu, S.; Bézard, J. Follicular fluid is not a compulsory carrier of the oocyte at ovulation in the mare. Equine Vet. J. 1997, 29, 22–24. [Google Scholar] [CrossRef] [PubMed]
Goudet, G.; Bezard, J.; Duchamp, G.; Palmer, E. Transfer of immature oocytes to a preovulatory follicle: An alternative to in vitro maturation in the mare? Equine Vet. J. 1997, 25, 54–59. [Google Scholar] [CrossRef]
Bashir, S.T.; Gastal, M.O.; Tazawa, S.P.; Tarso, S.G.S.; Hales, D.B.; Cuervo-Arango, J.; Baerwald, A.R.; Gastal, E.L. The mare as a model for luteinized unruptured follicle syndrome: Intrafollicular endocrine milieu. Reproduction 2016, 151, 271–283. [Google Scholar] [CrossRef]
Panelli, D.M.; Phillips, C.H.; Brady, P.C. Incidence, diagnosis and management of tubal and nontubal ectopic pregnancies: A review. Fertil. Res. Pr. 2015, 1, 15. [Google Scholar] [CrossRef]
Allen, W.R.; Wilsher, S.; Morris, L.; Crowhurst, J.S.; Hillyer, M.H.; Neal, H.N. Laparoscopic application of PGE2 to re-establish oviducal patency and fertility in infertile mares: A preliminary study. Equine Vet. J. 2010, 38, 454–459. [Google Scholar] [CrossRef] [PubMed]
Katila, T.; Reilas, T.; Nivola, K.; Peltonen, T.; Virtala, A.-M. A 15-year survey of reproductive efficiency of Standardbred and Finnhorse trotters in Finland-descriptive results. Acta Vet. Scand. 2010, 11. [Google Scholar] [CrossRef]
Padmanabhan, V.; Veiga-Lopez, A. Sheep models of polycystic ovary syndrome phenotype. Mol. Cell. Endocrinol. 2013, 373, 8–20. [Google Scholar] [CrossRef] [PubMed]
Bourgneuf, C.; Bailbé, D.; Lamazière, A.; Dupont, C.; Moldes, M.; Farabos, D.; Roblot, N.; Gauthier, C.; Mathieu d’Argent, E.; Cohen-Tannoudji, J.; et al. The Goto-Kakizaki rat is a spontaneous prototypical rodent model of polycystic ovary syndrome. Nat. Commun. 2021, 12, 1064. [Google Scholar] [CrossRef]
Publisher’s Note: MDPI stays neutral with regard to jurisdictional claims in published maps and institutional affiliations.
© 2021 by the authors. Licensee MDPI, Basel, Switzerland. This article is an open access article distributed under the terms and conditions of the Creative Commons Attribution (CC BY) license (https://creativecommons.org/licenses/by/4.0/).
https://www.mdpi.com/2076-2615/11/8/2304/htm
https://www.researchgate.net/figure/Anatomy-of-the-equine-ovary-in-a-fillies-and-b-adults-visualised-by-H-E-staining_fig1_272515862
Abstract
Characterization of the ovarian preantral follicle population is a necessary step to improve understanding of folliculogenesis and ovarian physiology. Therefore, in the present study, the preantral follicle population in the equine ovary in young and old mares was investigated according to follicular morphology, follicular class, distance from the geometric center using ovarian maps, and follicular density within ovarian portions (lateral vs intermediary) and regions (dorsal vs ventral). Ovaries were collected from an abattoir and histologically processed for evaluation, and the follicle population was calculated. Overall, in the current detailed study, a higher preantral follicle population per mare ovary (mean: 82,206 ± 50,022; range: 1477 to 773,091) than originally reported was identified. Additionally, a mare age effect was observed in the follicle population (young: 152,664 vs old: 11,750) and the spatial distribution of morphologically normal and abnormal follicles and the density and population of follicular classes. These results demonstrate that, in addition to the preantral follicle population in the mare ovary being comparable to that of other species, the location and spatial distribution of these follicles is dynamic and varies depending on mare age and follicle status (i.e. morphology and developmental stage). The characterization of the distribution and population of preantral follicles in the mare ovary provided by this study can potentially aid in improving reproductive studies and assisted reproductive techniques and may expand the understanding of mechanisms involving ovarian plasticity and follicular migration.
Lay summary
Knowledge of the distribution and population of immature eggs within follicles (preantral follicles) in the ovaries of mares can improve approaches to assisted reproductive techniques and fertility preservation. As the existing research on horse preantral follicle population was focused solely on large follicles, the present study provides an updated investigation of small and large preantral follicles in the mare, showing that the population is similar to those in other species. This study also shows that the way these follicles are distributed in the ovary varies depending on age and follicle characteristics. Results from this study may help to highlight which areas of the mare ovary should be looked at to find samples of good-quality follicles.
Keywords: equine ovarian plasticity; preantral follicles; spatial distribution and population; folliculogenesis
Introduction
At birth in most species, a finite pool of preantral follicles exists in the ovaries of females (Kezele et al.2002), which characterizes the main oocyte reserve of a given individual. Studies aiming to characterize the population of preantral follicles in the ovary are of great scientific value, as these can aid in increasing physiological knowledge about folliculogenesis, a critical concept for optimizing female fertility treatments and assisted reproductive techniques (ARTs).
In this context, the mare is a particularly appealing model to study folliculogenesis and follicle population, due to shared similarities with both women and other livestock species, making the mare a valuable dual-purpose, dual-benefit animal model (for review, see Carnevale 2008, Mihm & Evans 2008, Ginther 2012, Carnevale et al. 2020, Gastal et al. 2020, Benammar et al. 2021). Studies assessing preantral follicle population have been conducted in jennies (Lopes et al.2017), ewes (Amorim et al. 2000), does (Lucci et al.1999), cows (Lucci et al. 2002, Silva-Santos et al. 2011), gilts (Alves et al. 2012), and women (Gougeon & Chainy 1987). In mares, however, the only study in which follicular population was assessed counted preantral follicles (mean per ovary: 35,000; range per ovary: 6400–75,200) greater than 50 µm in diameter in young (2–4 years) mares (Driancourt et al. 1982). Nevertheless, it has been shown in recent studies that equine primordial, transitional, and primary preantral follicles have diameters smaller than 50 µm (Haag et al. 2013, Alves et al.2015). Thus, the original work assessing equine preantral follicle population (Driancourt et al. 1982) may have underestimated the number of follicles per ovary and, therefore, warrants an in-depth, updated study.
In addition to quantification of the follicular population in the mare ovary, the effects of age and supportive techniques used to evaluate preantral follicles (i.e. follicular morphology and classification, spatial distribution, and density) should simultaneously be characterized. The complex events of follicular development and migration are not uniform within the ovary (Riley et al. 2001, Faire et al. 2015). This leads to heterogeneity of the follicular population and large variation in the numbers and classes of follicles harvested in different samples of ovarian tissue in the mare (Alves et al.2016, Gastal et al.2017a) and several other species (woman: Schmidt et al. 2003, Kristensen et al. 2011; cow: Silva-Santos et al. 2011; ewe: Fransolet et al. 2014; doe: Brandão et al.2018; deer: Gastal et al. 2017b; mouse: Dath et al.2010, Malki et al.2015). This follicular heterogeneity and variation can be explained by the dynamic ovarian plasticity that has been suggested to occur in women and mares (Woodruff & Shea 2011, Alves et al. 2018). In fact, Alves et al. (2018) evaluated ovarian portions (lateral and intermediary) and regions (dorsal and ventral) in detail, considering the spatial distribution of preantral follicles according to mare age and follicle class. However, the morphology of preantral follicles across the whole ovary in different regions and portions according to mare age has not been evaluated. Thus, a novel study that combines an in-depth characterization of the follicle population with the distribution of morphologically normal follicles according to mare age is crucial.
The aims of this study were to assess the population of equine preantral follicles in young and old mares according to (i) follicular morphology, (ii) follicular class, (iii) distance from the ovarian geometric center, and (iv) follicular density within ovarian portions (lateral vs intermediary) and regions (dorsal vs ventral).
Materials and methods
Ovaries
Ovaries were harvested during the physiological breeding season from mixed-breed, light-horse mares (n = 8) at an equine abattoir located in Brazil (30°20’38”S, 54°20’31”W) and separated into two age groups (young: 4–9 years and old: ≥20 years; n = 4 pairs of ovaries for each group) based upon dental characteristics. Immediately after slaughter, each ovary was divided into three longitudinal portions: n = 2 lateral portions and n = 1 intermediary portion (Fig. 1A). Afterward, each ovarian portion was immediately fixed in 4% paraformaldehyde for 24 h and placed in 70% alcohol until histological processing. None of the ovaries from the eight mares contained visible preovulatory follicles and/or corpora lutea. Reproductive status (anestrus or cycling) of the mares was unknown.

Figure 1
Illustration of experimental procedures performed to assess preantral follicle morphology, classification, spatial distribution, density, and population in the equine ovary. Ovaries were divided into (A) three portions (lateral, n = 2; intermediary, n = 1), followed by (B) histological processing and sectioning to allow for evaluation of follicle density and population. The whole follicle population was estimated per ovary using a formula, as previously described (Gougeon & Chainy 1987, Gastal et al.2017b). (C, D, and E) A square grid sheet with columns and rows represented by letters and numbers, respectively, was placed upon the histological slides for microscopic evaluation. (D) Geometric center was determined for each histological section. (E) Analysis of preantral follicle distribution (distance in mm and angle in relation to the geometric center) and determination of dorsal and ventral regions of the ovary; five representative histological sections per ovarian portion per mare, now termed ‘ovarian maps’ (n = 5 maps per portion; 15 maps per ovary; 240 maps total for all 16 ovaries from 8 mares), were made. Representative histological sections depicting (F and G) lower and (I and J) higher follicle (primordial, transitional, and primary) density per microscopic field are shown in low magnification. (H) Normal and (K) abnormal late secondary follicles are shown in high magnification. (F, G, I, and J) Scale bars = 100 µm, magnification = ×200; and (H and K) scale bars = 50 µm, magnification = ×400. (A–E) Diagrams adapted from Alves et al. (2018).
Citation: Reproduction and Fertility 3, 2; 10.1530/RAF-21-0100Download Figure
Download figure as PowerPoint slide
Histological processing
All ovarian portions were dehydrated, embedded in paraffin wax, and completely cut into 7 μm serial sections (Alves et al. 2015). To avoid double counting of follicles, and considering the frequency and diameter of equine primordial and transitional follicles as reported by Alves et al. (2015), every fifth section of the ovary was mounted onto large (127 × 102 mm) microscope slides (Fig. 1B). To ensure good tissue quality for the analyses, only histological sections with clearly visible borders and intact ovulation fossa without lacerations were chosen. Slides were stained using periodic acid-schiff and counterstained with hematoxylin, then prepared for spatial distribution evaluation.
Preparation for preantral follicle spatial distribution evaluation
The preparation of slides for determination of the spatial distribution for preantral follicles in the ovarian portions and regions was performed as previously described (Alves et al. 2018):
A square grid sheet (area of each square = 0.0625 cm2) with rows (indicated by numbers) and columns (indicated by letters) was designed and printed on an overhead transparency sheet at the same dimensions of the microscope slides.
Histological sections were overlaid with the square grid sheet (Fig. 1C) and scanned using a photo editing program (Adobe Photoshop CS4; San Jose, USA). All histological sections were scanned with the ovulation fossa positioned at the bottom and the square grid sheet aligned to the upper-left corner of the slide. These scanned, digital images were used as locating guides for the microscopic evaluation of the spatial distribution of preantral follicles.
Subsequently, the geometric center of each digitally scanned histological section was defined. Thirty equidistant points throughout the perimeter of each histological section were determined (Adobe Photoshop CS4; Fig. 1D). The distance of each point relative to the X- and Y-axes was recorded, and the geometric center was calculated using the following formulas:


Geometric center = mean distance (X;Y)
After determining the geometric center of each histological section, a longitudinal line was made using a marker tool (Adobe Photoshop CS4), and the ovarian regions above and below the longitudinal line were termed the dorsal and ventral regions, respectively.
Finally, five representative histological sections, from now on referred to as ‘ovarian maps’, per portion per ovary for each mare were used to assess preantral follicle distance from the geometric center (Fig. 1E).
Microscopic evaluation
The histological sections were analyzed using light microscopy (Nikon E200; Tokyo, Japan) at ×400 magnification using an image capture system (Leica Imaging Software). For each ovarian portion, the following end points were evaluated considering preantral follicles: morphology, class, spatial distribution (concerning the ovarian region and distance from the geometric center), density, and population.
Preantral follicle morphology and classification
Regarding morphology, preantral follicles were classified as normal (oocyte nucleus showing no signs of pyknosis, and the ooplasm surrounded by well-organized granulosa cells) or abnormal (oocyte showing a pyknotic nucleus or a retracted ooplasm with detachment or disorganization of the granulosa cells), as previously described (Alves et al. 2015; Fig. 1H and K). Only preantral follicles with a visible oocyte nucleus were counted and classified according to developmental class as primordial (oocyte surrounded by a single layer of flattened granulosa cells), transitional (oocyte surrounded by a single layer of both flattened and cuboidal granulosa cells), primary (oocyte surrounded by a single layer of cuboidal granulosa cells), or secondary (oocyte surrounded by two or more layers of cuboidal granulosa cells), as previously described (Alves et al. 2015).
Measurement of distance from geometric center
Distance (mm) and angle (0º–360º) of the preantral follicles within each ovarian map were measured in relation to the geometric center using a ruler tool in the imaging software (Adobe Photoshop CS4). Polar plots using distance from the geometric center and angle (r, θ) coordinates were generated, as performed by Alves et al. (2018).
Density determination
Regarding preantral follicle density, the perimeter of scanned images of each histological section was delimited using a photo editing program (Adobe Photoshop CS4) and the scale-calibrated area was measured in cm2. Afterward, follicular density (Fig. 1F, G, I and J) was calculated with the following formula: follicular density = number of follicles observed/area of the histological section (cm2).
Population estimation
To determine the preantral follicle population, the oocyte nucleus was measured and used as a marker, as previously described (Gougeon & Chainy 1987). Then, the population was calculated using the formula Nt = (No × St × ts)/(So × do), where Nt = total calculated number of follicles of a class; No = number of follicles observed in the whole ovary; St = total number of ovarian sections made; ts = thickness of each ovarian section (µm); So = total number of sections evaluated; and do = mean oocyte nucleus diameter of each follicle class (Gougeon & Chainy 1987, Gastal et al. 2017b). Counting and classification of preantral follicles were performed by K A Alves, while follicle spatial distribution and population were calculated by B G Alves.
Statistical analysis
Statistical analyses were performed using Sigma Plot, version 11.0 (Systat Software Inc., EUA). Data that were determined to be non-normally distributed using the Kolmogorov-Smirnov test (distance from geometric center, density, and population) were transformed using base 10 logarithm (Log10). One young mare (#2; Table 1) was found to be a statistical outlier in a few end points. This finding is relatively common in nature due to high incidences of follicular heterogeneity and individual variation; thus, this mare was intentionally kept in the data set to model what is naturally observed in several mammalian species (mare: Alves et al. 2016; woman: Kristensen et al. 2011; cow: Silva-Santos et al. 2011; doe: Brandão et al.2018; deer: Gastal et al. 2017b; mouse: Malki et al.2015). However, to account for the outlier status of this mare’s data, transformations or rank-based statistical tests were used. To compare mean values between groups for the follicle end points morphology, classification, distance from geometric center, density, and population, a two-way ANOVA followed by post hoc Tukey’s test, t-test, or Wilcoxon–Mann–Whitney test were used. Percentages of morphologically normal preantral follicles were assessed using chi-square or Fisher’s exact tests. Data are presented as number, mean ± s.e.m., and percentage. Statistical significance was defined as P < 0.05 (two-sided), and P ≥0.05 and ≤ 0.1 indicated a tendency to differ.
Table 1
Number of normal and abnormal preantral follicles observed for each classification in collected ovarian tissue per mare. A total of 438 histological sections were read (55 ± 3.1 sections per ovarian pair of each mare). Mares 1, 2, 3, and 4 were 8, 4, 4, and 9 years old, respectively. Mares 5–8 were all ≥20 years old.
AnimalPrimordialTransitionalPrimarySecondaryOverall
NormalAbnormalNormalAbnormalNormalAbnormalNormalAbnormalNormalAbnormal1 307 17 35 3 6 0 5 0 353 20
2 2679 764 3777 866 2486 419 3 1 8945 2050
3 247 34 147 13 55 2 3 0 452 49
4 233 49 60 8 20 0 2 0 315 57
5 468 6 116 7 14 3 2 0 600 16
6 81 4 32 2 10 2 2 1 125 9
7 58 3 2 4 3 1 1 0 64 8
8 56 5 16 4 7 3 0 0 79 12
Overall 4129 882 4185 907 2601 430 18 2 10,933 2221
Results
Follicular morphology among follicular classes
A total of 438 ovarian histological sections were evaluated (27 ± 1.2 sections per ovary) and 13,154 preantral follicles were recorded in the lateral (n = 6399) and intermediary (n = 6755) ovarian portions. The number of preantral follicles (Table 1) observed per follicular class and morphological classification for each individual mare are shown. The overall number of preantral follicles observed per mare revealed a wide range (normal: 64–8945; abnormal: 8–2050); mare #7 had the lowest number of both normal and abnormal follicles, while mare #2 showed the highest. The mean (± s.e.m.) number and percentages of normal follicles (Fig. 2) per follicular class were also demonstrated between age groups and overall. Young mares had more (P < 0.05; Fig. 2A) normal follicles than old mares, regardless of follicular class (primordial, transitional, primary, secondary) and overall. Considering the mean number of normal preantral follicles regardless of age group (Fig. 2B), fewer (P < 0.05) normal secondary follicles were observed compared to early preantral follicular classes (i.e. primordial and transitional). No differences (P > 0.05) were observed between the mean number of primary follicles compared to the other follicular classes. Concerning the percentage of preantral follicles between age groups (Fig. 2C) in young mares, the percentage of normal follicles increased (P < 0.05) progressively until the primary follicular class. In contrast, old mares followed an opposite trend, whereby the percentage of normal follicles decreased (P < 0.05) until the primary follicular class. When comparing age groups, old mares had higher (P < 0.05) percentages of normal primordial, transitional, and overall follicles than young mares. Meanwhile, when age groups were pooled, the percentage of normal follicles (Fig. 2D) did not differ (P > 0.05).

Figure 2
(A and B) Mean (± s.e.m.) number of preantral follicles counted and (C and D) percentage of normal preantral follicles observed in (A and C) young and old mares and (B and D) with age groups combined. *Indicates that within the same follicle class, values between age groups differed (P< 0.05). A,BRegardless of mare age, values between follicle classes without a common superscript differed (P < 0.05). a,b,cWithin the young age group, values without a common superscript differed (P < 0.05). X,Y,ZWithin the old age group, values without a common superscript differed (P< 0.05). No difference (P > 0.05) was observed for the percentage of normal follicles between classes. (A) Below the break, Y-axis scale is every 5, and above the break, Y-axis scale changes to every 1000. (B) Below the break, Y-axis scale is every 2, and above the break, Y-axis scale changes to every 500. (C and D) Y-axis scale remains the same below and above the break.
Citation: Reproduction and Fertility 3, 2; 10.1530/RAF-21-0100Download Figure
Download figure as PowerPoint slide
Preantral follicle distance from the ovarian geometric center
A total of 240 ovarian maps were evaluated (15 per ovary), and 9284 preantral follicles were recorded in the lateral (n = 4901) and intermediary (n = 4383) portions and the dorsal (n = 5374) and ventral (n = 3910) regions. The mean distance of the observed preantral follicles in relation to the ovarian geometric center was determined considering the different ovarian portions and regions for both normal and abnormal follicles (Table 2). With regard to morphological classification (i.e. normal vs abnormal), the normal follicles within the lateral portion and ventral region were closer (P < 0.05) to the geometric center than were the abnormal follicles within the same portion and region. Meanwhile, in the intermediary portion, the normal follicles within both regions were farther (P < 0.05) from the geometric center compared to the abnormal follicles. When ovarian regions were combined, the normal and abnormal follicles in the lateral portion did not differ (P > 0.05) in distance from the geometric center; however, in the intermediary portion, normal follicles were farther (P< 0.05) than abnormal follicles from the geometric center. Within the whole ovary, normal and abnormal follicles did not differ (P > 0.05) regarding distance from the geometric center. Follicles in the dorsal region were closer (P< 0.05) to the geometric center than were follicles in the ventral region, regardless of ovarian portion and morphological classification. When comparing ovarian portions within the dorsal region, follicles in the lateral portion were farther (P < 0.05) from the geometric center than were those in the intermediary portion, regardless of follicular morphology. However, in the ventral region and in the regions combined, only normal follicles in the lateral portion were closer (P < 0.05) to the geometric center. Representative polar plots (Fig. 3) considering the distance of normal (Fig. 3A and C) and abnormal (Fig. 3B and C) follicles in regard to the geometric center in the lateral (Fig. 3A and B) and intermediary (Fig. 3C and D) ovarian portions are shown. All mares (n = 8) were considered for each polar plot, with each mare represented using a different color.

Figure 3
Polar plots depicting distance from the ovarian geometric center of individual follicles separated by morphological classification as (A and C) normal and (B and D) abnormal and ovarian portions as (A and B) lateral and (C and D) intermediary. Follicles from each mare (n = 8) are indicated by different colors. Polar coordinates (r, θ) were determined using the distance from the geometric center (mm; r) and angulation data (°; θ) of the follicles recorded in ovarian maps. Only histological sections with clear borders and intact ovulation fossa without lacerations were chosen to be used for ovarian mapping (n = 5 maps per portion; 15 maps per ovary; 240 maps total for all 16 ovaries from 8 mares). Ovarian regions were determined based upon the 180° midline; the region above is dorsal (180°–0°), and the region below is ventral (181°–360°).
Citation: Reproduction and Fertility 3, 2; 10.1530/RAF-21-0100Download Figure
Download figure as PowerPoint slide
Table 2
Mean (± s.e.m.) distance to the geometric center (mm) of normal and abnormal equine preantral follicles according to different ovarian portions (lateral and intermediary) and regions (dorsal and ventral).
Ovarian regionsOvarian portion§
LateralIntermediaryCombined portions
NormalAbnormalNormalAbnormalNormalAbnormalDorsal (n = 5374) † 5.54 ± 0.05aAX 5.38 ± 0.11aAX 5.16 ± 0.05aAY 4.68 ± 0.14bAY 5.36 ± 0.04aA 5.15 ± 0.08aA
Ventral (n = 3910) 5.60 ± 0.08aBX 6.01 ± 0.19bBX 7.22 ± 0.08aBY 6.07 ± 0.14bBX 6.37 ± 0.06aB 6.04 ± 0.12aB
Combined regions 5.57 ± 0.04aX 5.61 ± 0.10aX 6.01 ± 0.05aY 5.37 ± 0.10bX 5.78 ± 0.03a 5.52 ± 0.07a
§Ovaries were divided longitudinally into three portions: two lateral and one intermediary. Five representative ovarian maps were evaluated per ovarian portion (n = 15 maps per ovary, n = 240 maps in total). Only histological sections with clear borders and intact ovulation fossa, without lacerations, were chosen for ovarian mapping. †Number of preantral follicles of all classes (primordial, transitional, primary, secondary) evaluated per ovarian region. a,bBetween morphological classifications and within each ovarian portion and region, values without a common superscript differed (P < 0.05). A,BBetween ovarian regions and within each ovarian portion and morphological classification, values without a common superscript differed (P < 0.05). X,YBetween ovarian portions and within each ovarian region and morphological classification, values without a common superscript differed (P < 0.05).
Preantral follicle density considering different ovarian portions, regions, and age groups
The mean density of preantral follicles according to mare age evaluated in different ovarian portions throughout the ovarian regions and follicle class is shown (Fig. 4). Within the same ovarian portion and between age groups (Fig. 4A), young mares had higher (P < 0.05) densities of preantral follicles than old mares, regardless of region of the ovary. Additionally, when the lateral and intermediary portions were combined (overall analysis), young mares also showed greater (P < 0.05) follicular densities than old mares, regardless of ovarian region. In the dorsal regions of young mares, a higher (P < 0.05) density of preantral follicles was observed in the lateral portion compared to the intermediary portion; however, an opposite trend was observed in the old mares with higher (P< 0.05) densities in the intermediary portion. In the ventral regions of both young and old mares, the intermediary portion had higher (P < 0.05) follicle densities compared to the lateral portion. Furthermore, the mean preantral follicle densities according to different follicular classes and age groups were evaluated in different ovarian portions (Fig. 4B). For statistical purposes, primordial and transitional follicles were combined (early preantral), as were primary and secondary follicles (late preantral). Regardless of portions and follicular classes, as well as in the overall analysis, young mares had higher (P < 0.05) densities of follicles when compared to old mares. For the young mares, the intermediary portion had higher (P < 0.05) densities for both follicular classes than the lateral portion, while old mares showed higher (P < 0.05) density of early preantral follicles only in the intermediary portion. As expected, regardless of age group and ovarian portion, the densities of early preantral follicles were higher (P< 0.05) than those of late preantral follicles.

Figure 4
(A) Mean (± s.e.m.) preantral follicle density, regardless of follicle class, observed in different ovarian portions (lateral and intermediary), and overall, within regions (dorsal and ventral) in young (4–9 years) and old (≥20 years) mares. (B) Mean (± s.e.m.) preantral follicle density per follicle class (early preantral follicles: primordial and transitional vs late preantral follicles: primary and secondary) observed in each ovarian portion, and overall, in young (4–9 years) and old (≥20 years) mares, regardless of ovarian region. The ovaries were divided longitudinally into three portions: two lateral and one intermediary, for a total of 438 histological sections (n = 277 lateral sections, n = 161 intermediary sections). *Indicates young mares had greater mean follicle density than old mares within the same ovarian portion and overall. A,BWithin the (A) dorsal region or (B) early preantral follicle class, and within the same age group, values without a common superscript between ovarian portions differed (P < 0.05). X,YWithin the (A) ventral region or (B) late preantral follicle class, and within the same age group, values without a common superscript between ovarian portions differed (P < 0.05). In the overall analyses, within each age group, the follicle densities between (A) ovarian regions and between (B) follicle classes did not differ (P > 0.05) when the lateral and intermediary ovarian portions were combined. No differences (P> 0.05) were observed in follicle densities between (A) regions and (B) follicle classes within the same portion and age group. (A and B) Below the break, Y-axis scale is every 0.2, and above the break, Y-axis scale changes to every 5.
Citation: Reproduction and Fertility 3, 2; 10.1530/RAF-21-0100Download Figure
Download figure as PowerPoint slide
Preantral follicle population according to age group and follicular classes
The mean population of preantral follicles between young and old mares, considering different follicular classes, is shown (Table 3). Primordial and transitional follicles were combined (early preantral) for statistical purposes. Within the same follicular class, the population of early preantral follicles in young mares was higher (P < 0.05) than in old mares; however, for late preantral follicles (primary and secondary), no differences (P > 0.05) were observed between ages. Furthermore, regardless of class, the overall follicular population in young mares was greater (P < 0.05) than in old mares. Within each age group, and when ages were combined, the follicular population decreased (P < 0.05) considering more advanced classes.
Table 3
Mean (± s.e.m.) equine preantral follicular population estimated per ovary according to age group and follicular class.
Age group (years)Early preantral†PrimarySecondaryOverall4–9
Mean ± s.e.m. 110,846.6 ± 63,360.1aA 42,019.5 ± 35,104.7bA 230.6 ± 83.1cA 152,663.5 ± 96,345.9A
Range 5307–489,793 112–285,264 0–580 5778–773,091
≥20
Mean ± s.e.m. 11,038.2 ± 4688.1aB 628.3 ± 147.7bA 100.5 ± 54.8cA 11,749.8 ± 4802.3B
Range 1363–38,556 112–1,075 0–377 1477–39,874
Combined ages
Mean ± s.e.m. 60,942.4 ± 33,284.7a 21,323.9 ± 17,779.3b 165.5 ± 50.9c 82,206.6 ± 50,022.4
Range 1363–489,793 112–285,264 0–580 1477–773,091
A,BWithin the same follicular class, and overall, values without a common superscript differed (P < 0.05). a,b,cWithin the same age group, and combined, values without a common superscript differed (P< 0.05). †Data from primordial and transitional follicles were combined. Four mares were included in each age group (eight mares total).
Follicle population considering age groups, ovarian portions, and follicular classes
The populations of preantral follicles between age groups, ovarian portions, and follicular classes are shown (Fig. 5), with primordial and transitional follicles combined (early preantral; Fig. 5A). With respect to the ovarian portions, a different pattern of follicle population was observed according to the age groups. In young mares, the lateral portion showed decreasing (P < 0.05) follicular populations between every follicle class, from early preantral to advanced classes. In the intermediary portion of young mares, the population decreased (P < 0.05) between early preantral and late preantral classes but lacked statistical difference (P > 0.05) between the primary and secondary classes. Interestingly, old mares showed the opposite pattern, with a steady population (P > 0.05) between primary and secondary classes in the lateral portion and a constant decrease (P < 0.05) between every follicle class in the intermediary portion. When comparing follicular populations between age groups, only the lateral portion of young mares tended (P = 0.06) to have a higher early preantral follicle population. Once follicle classes were combined (Fig. 5B), the population in the lateral portion was greater (P < 0.05) in young mares than in old mares; nevertheless, no difference (P > 0.05) was observed within the intermediary portions, potentially due to the variability between individuals demonstrated by the large error bars.

Figure 5
Mean (± s.e.m.) equine preantral follicle population estimated in different ovarian portions (lateral and intermediary) (A) per follicle class and with (B) follicle classes combined. a,b,cWithin the young age group and same ovarian portion, values without a common superscript differed (P < 0.05) between follicle classes. X,Y,ZWithin the old age group and same ovarian portion, values without a common superscript differed (P < 0.05) between follicle classes. #Indicates tendency (P = 0.06) to differ between age groups within ovarian portions and follicular classes; no further differences (P > 0.05) were observed. No differences (P > 0.05) were observed between portions within each age group and the same follicular class. A,BWhen follicle classes were combined, within each ovarian portion, values without a common superscript differed (P < 0.05). (A) Below the break, Y-axis scale is every 100, and above the break, Y-axis scale changes to every 20,000. (B) Below the break, Y-axis scale is every 5,000, and above the break, Y-axis scale changes to every 20,000.
Citation: Reproduction and Fertility 3, 2; 10.1530/RAF-21-0100Download Figure
Download figure as PowerPoint slide
Discussion
The present study quantified, for the first time in mares, the preantral follicle population including follicles smaller than 50 µm in diameter (i.e. primordial, transitional, and primary) via histological and mathematical methods. Another original aspect of this work was the evaluation of the spatial distribution of preantral follicles considering morphology and the influences of mare age. The main findings of the present study demonstrated that mares have a preantral follicle population higher than previously reported (Driancourt et al. 1982) and similar to that of other livestock species and women. Another novel finding demonstrated that the follicular spatial distribution within the portions and regions of the ovary changes depending upon follicle morphology and class and mare age. Finally, large individual differences (variation between mares) and heterogeneity (variation between samples from the same mare) in follicle population and spatial distribution were observed.
In the present study, the overall preantral follicle population per ovary, regardless of age groups, had a higher-than-expected population compared to a previous classical report (35,000 follicles in Driancourt et al.1982 vs 82,000 in the present study). We believe this discrepancy in follicle population is due to the fact that Driancourt et al. (1982) counted only follicles that were greater than 50 µm in diameter. Importantly, equine primordial, transitional, and primary follicles have diameters smaller than 50 µm and compose a large percentage of the follicular reserve (Haag et al. 2013, Alves et al. 2015). For all mares in the current study, the highest numbers of follicles observed belonged to the primordial (5011 follicles, 38%) and transitional (5092 follicles, 39%) classes, and these numbers (from a total of 13,154 follicles) were included to mathematically estimate the overall ovarian population. Therefore, Driancourt et al. (1982) may have underestimated the follicular population of the mare by at least 50%. Another novel finding in this study was that young mares had a greater preantral follicle population (152,664 follicles) than old mares (11,750 follicles), a characteristic that is similar to other species (women: Gougeon & Chainy 1987, Gleicher & Barad 2011; bovine: Malhi et al. 2005; macaques: Nichols et al. 2005; deer: Gastal et al. 2017b). In addition to the updated equine preantral follicle population reported in the current study (82,206 ± 50,022), a large variation in the population of follicles per ovary was calculated (range: 1466–773,091). This large variation is comparable to findings in other species such as cows (ranges: 59,798–78,820, Lucci et al. 2002; 39,438–89,577, Silva-Santos et al. 2011), ewes (range: 7333–44,633, Amorim et al. 2000), gilts (range: 67,599–291,898, Alves et al. 2012), does (range: 20,122–80,739, Lucci et al.1999), and women (range: 2700–79,600, Gougeon & Chainy 1987). This fact leads us to assume that the mare, despite having some unique anatomical differences (i.e. a single point where all ovulations occur called the ovulation fossa and inverted follicular layers where the ovarian cortex makes up the center of the ovary while the medulla surrounds the cortex) compared to other species, could continue to be an alternative animal model for dual-purpose, dual-benefit studies considering ARTs (Benammar et al. 2021).
An interesting effect of age on the populations of preantral follicles in the intermediary and lateral portions was found. To the best of our knowledge, there is no report in the literature that has evaluated the population of preantral follicles regarding spatial distribution in any species. The reason for the different patterns in the populations of preantral follicle classes between portions within each age group observed in this study is unknown and deserves further investigation.
In the present study, large individual differences and follicular heterogeneity were characterized for each end point evaluated (i.e. follicle number, distance from geometric center, density, and population). Extensive follicular heterogeneity has been reported in mares (Alves et al. 2017, 2018, Gonzales et al.2017) as well as other livestock species (cows: Aerts et al. 2008, Silva-Santos et al.2011; ewes: Fransolet et al. 2014) and women (Fabbri et al. 2012). This follicular heterogeneity and large differences between individuals and tissue samples may be due, at least in part, to the working hypothesis of ovarian plasticity (Woodruff & Shea 2011, Alves et al. 2018), which hypothesizes that preantral follicles migrate within the ovarian cortex during early folliculogenesis. Indeed, folliculogenesis encompasses a dynamic and complex process characterized by follicular quiescence (Faire et al.2015), activation, growth (Gaytan et al.2015), tissue remodeling of the ovarian stroma by antral follicles (Riley et al.2001), migration of preovulatory follicles toward the ovulation fossa (for review, see Gastal 2011) or atresia (Tatone et al. 2008). Altogether, the aforementioned events may lead to a heterogeneous and widely variable follicle population, both between and within individuals.
A core novel finding of the present study showed that follicle distance from the geometric center of the ovary in different portions and regions changes depending on morphology. While all follicles, regardless of morphology, within the dorsal region are closer to the geometric center than in the ventral region, morphologically normal follicles within the dorsal region and intermediary portion are farther from the geometric center than are abnormal follicles. These findings fit well into the working hypothesis proposed by Alves et al. (2018) that, as preantral follicles develop until the primary classification, they migrate closer to the geometric center. Once these follicles develop into secondary follicles, they begin to migrate farther from the geometric center. Thus, we hypothesize that, in the intermediary portion, once preantral follicles become abnormal, the migration process stops closer to the geometric center, while normal follicles will continue to migrate farther from the geometric center. These normal preantral follicles will later form the antral follicles randomly distributed throughout the ovarian cortex (Kimura et al. 2009, Alves et al.2018). This migration is potentially necessary for the continuation of folliculogenesis during the critical transition from preantral to antral follicle classes. To this end, studies that simultaneously evaluate the distance from the geometric center of follicles of differing classes in combination with morphology are warranted to validate our current working hypothesis.
The number of normal preantral follicles in the present study, as expected, was higher in young mares, regardless of class. This result is in accordance with previous reports, as aging is associated with significant decreases in the number of preantral follicles observed in the ovaries of livestock species (equine: Haag et al. 2013, Alves et al. 2017; bovine: Malhi et al. 2005), non-human primates (Nichols et al. 2005), and women (Gleicher & Barad 2011). Interestingly, despite having lower numbers of normal primordial and transitional follicles, old mares had higher percentages of these follicles than young mares in this study. These findings may be explained due to the fact that, during the lifespan of a female, a majority of follicles will begin to develop and leave the pool of quiescent follicles (Tatone et al. 2008). This process is potentially more intense in young females because of the large number of follicles that are available to grow. Once follicular growth has begun, only a tiny percentage of these preantral follicles will reach ovulation, with the rest undergoing atresia, decreasing the number of follicles within the ovary (Tatone et al. 2008) as a mare ages. The follicles that do remain in quiescence (primordial) or have likely started to grow (transitional) are potentially more resistant to atresia, as suggested by Aguiar et al. (2017). Thus, we speculate that, once a mare reaches old age, only the remaining primordial and transitional follicles are more often morphologically normal.
In terms of follicular density, the present study found that there is an age effect on the densities of preantral follicles in the different portions of the equine ovary. As expected, young mares had higher follicle densities for all ovarian portions and regions than old mares; however, different trends between portions were observed within age groups and regions. In this regard, the intermediary portion of old mares displayed similar follicle density between regions; meanwhile, a higher density of the early preantral follicle class was observed. Therefore, we hypothesize that the few remaining, atresia-resistant early preantral follicles of old mares tend to reside in the intermediary portion of the ovary, regardless of ovarian region. This lack of regional difference could potentially be explained by the fact that preantral follicles exhibit a more dispersive pattern as a mare reaches older age (Alves et al. 2018). Our hypothesis is further supported by the concept of ovarian plasticity (Woodruff & Shea 2011), as these early preantral follicles are still migrating toward the center of the ovary located in the intermediary portion. Regarding young mares, we postulate that the different trends of follicle density within the ovarian regions and higher densities of both follicle classes in the intermediary portion are potentially due to higher levels of follicular activity. For ovulation to occur in the mare, large antral/preovulatory follicles must develop and migrate toward the ovulation fossa within the ventral region of the ovary (Riley et al. 2001, Gastal 2011). Due to these facts, we hypothesize that, as these large follicles migrate toward the ovulation fossa, the much smaller preantral follicles are pushed into the dorsal region of the lateral portion in young mares. However, the intense follicular activity of young mares, translated by high numbers of early preantral and primary follicles migrating toward the ovarian geometric center (Alves et al. 2018), is potentially reflected in this study by the higher preantral follicle density in the ventral region of the intermediary portion. Considering that young mares have higher numbers of both preantral and antral follicles than old mares (Ginther et al.2008, 2009, Alves et al. 2017, Goncalves et al. 2020), our hypotheses may explain the different trends in follicular density observed in this study. In this aspect, future studies assessing follicle density of different classes, particularly late secondary and early antral follicles, in combination with morphology, portion, and regional location within the ovary, are appealing.
Overall, the present study reports for the first time (i) a higher preantral follicle population than originally reported by Driancourt et al. (1982), (ii) an effect of mare age on the spatial distribution of morphologically normal and abnormal follicles, (iii) an age effect on the density of follicular classes, and (iv) an effect of age on the population distribution of follicle classes. The in-depth characterization of the distribution and population of preantral follicles in the mare ovary provided by this study can aid in improving reproductive studies, ARTs, and procedures regarding mechanisms involving ovarian plasticity and follicular migration. Therefore, the application of results from this study may assist in targeting certain areas of the equine ovary to obtain higher follicular densities with better quality (i.e. morphologically normal) and particular classes. For example, if preservation of primordial follicles is desired in young equine ovarian tissue, the intermediary portion and ventral region of the ovary should be targeted for ovarian biopsy to harvest a high density of normal primordial follicles. Furthermore, using the information reported by the current study, we have provided working hypotheses that should be further explored to elucidate mechanisms related to ovarian physiology, folliculogenesis, and follicular migration.
Declaration of interest
The authors declare that there is no conflict of interest that could be perceived as prejudicing the impartiality of the research reported.
Funding
This work was supported by Southern Illinois University (SIU), Carbondale, IL, USA. K A Alves and G D A Gastal were recipients of PhD scholarships and B G Alves was the recipient of a post-doctoral fellowship from the National Council for Scientific and Technological Development (CNPq). Funders had no role in study design, data collection and analysis, decision to publish, or preparation of this manuscript.
Author contribution statement
Conceived and designed the experiment: B G A, K A A, G D A G, and E L G. Performed the experiment: B G A, K A A, G D A G, and E L G. Analyzed the data and prepared figures: K A H, F L N A, B G A, K A A, M O G, and E L G. Contributed reagents/materials/analysis tools: E L G. Wrote the paper: K A H, F L N A, and E L G.
Acknowledgements
The authors would like to thank M P B Linardakis and the Floresta Ltda. abbatoir for allowing collection of the ovaries. Research was supported by Southern Illinois University (SIU), Carbondale, IL, USA, the Coordination for the Improvement of Higher Education Personnel (CAPES), and the National Council for Scientific and Technological Development (CNPq).
References
Aerts JMJ, Martinez-Madrid B, Flothmann K, De Clercq JBP, Van Aelst S & Bols PEJ 2008Quantification and viability assessment of isolated bovine primordial and primary ovarian follicles retrieved through a standardized biopsy pick-up procedure. Reproduction in Domestic Animals 43360–366. (https://doi.org/10.1111/j.1439-0531.2007.00915.x)Search Google Scholar
Export Citation
Aguiar FLN, Lunardi FO, Lima LF, Bruno JB, Alves BG, Magalhães-Padilha DM, Cibin FWS, Berioni L, Apgar GA & Lo Turco EG et al.2017 Role of EGF on in situ culture of equine preantral follicles and metabolomics profile. Research in Veterinary Science 115 155–164. (https://doi.org/10.1016/j.rvsc.2017.04.001)Search Google Scholar
Export Citation
Alves BG, Alves KA, Araújo VR, Beletti ME, Gambarini ML & Jacomini JO 2012 Quantitative and morphological study of preantral follicles from prepubertal gilts. Acta Scientiae Veterinariae 40 1079.Search Google Scholar
Export Citation
Alves KA, Alves BG, Rocha CD, Visonná M, Mohallem RFF, Gastal MO, Jacomini JO, Beletti ME, Figueiredo JR & Gambarini ML et al.2015 Number and density of equine preantral follicles in different ovarian histological section thicknesses. Theriogenology 83 1048–1055. (https://doi.org/10.1016/j.theriogenology.2014.12.004)Search Google Scholar
Export Citation
Alves KA, Alves BG, Gastal GDA, de Tarso SGS, Gastal MO, Figueiredo JR, Gambarini ML & GastalEL 2016 The mare model to study the effects of ovarian dynamics on preantral follicle features. PLoS ONE 11 e0149693. (https://doi.org/10.1371/journal.pone.0149693)Search Google Scholar
Export Citation
Alves KA, Alves BG, Gastal GDA, Haag KT, Gastal MO, Figueiredo JR, Gambarini ML & Gastal EL2017 Preantral follicle density in ovarian biopsy fragments and effects of mare age. Reproduction, Fertility, and Development 29 867–875. (https://doi.org/10.1071/RD15402)Search Google Scholar
Export Citation
Alves BG, Alves KA, Gastal GDA, Gastal MO, Figueiredo JR & Gastal EL 2018 Spatial distribution of preantral follicles in the equine ovary. PLoS ONE 13 e0198108. (https://doi.org/10.1371/journal.pone.0198108)Search Google Scholar
Export Citation
Amorim CA, Rodrigues APR, Lucci CM, Figueiredo JR & Gonçalves PBD 2000 Effect of sectioning on the number of isolated ovine preantral follicles. Small Ruminant Research 37 269–277. (https://doi.org/10.1016/s0921-4488(9900153-4)Search Google Scholar
Export Citation
Benammar A, Derisoud E, Vialard F, Palmer E, Ayoubi JM, Poulain M & Chavatte-Palmer P 2021 The mare: a pertinent model for human assisted reproductive technologies? Animals 11 2304. (https://doi.org/10.3390/ani11082304)Search Google Scholar
Export Citation
Brandão FAS, Alves BG, Alves KA, Souza SS, Silva YP, Freitas VJF, Teixeira DIA & Gastal EL 2018Laparoscopic ovarian biopsy pick-up method for goats. Theriogenology 107 219–225. (https://doi.org/10.1016/j.theriogenology.2017.10.042)Search Google Scholar
Export Citation
Carnevale EM 2008 The mare model for follicular maturation and reproductive aging in the woman. Theriogenology 69 23–30. (https://doi.org/10.1016/j.theriogenology.2007.09.011)Search Google Scholar
Export Citation
Carnevale EM, Catandi GD & Fresa K 2020 Equine aging and the oocyte: a potential model for reproductive aging in women. Journal of Equine Veterinary Science 89 103022. (https://doi.org/10.1016/j.jevs.2020.103022)Search Google Scholar
Export Citation
Dath C, Van Eyck AS, Dolmans MM, Romeu L, Delle Vigne L, Donnez J & Van Langendonckt A 2010Xenotransplantation of human ovarian tissue to nude mice: comparison between four grafting sites. Human Reproduction 25 1734–1743. (https://doi.org/10.1093/humrep/deq131)Search Google Scholar
Export Citation
Driancourt MA, Paris A, Roux C, Mariana JC & Palmer E 1982 Ovarian follicular populations in pony and saddle-type mares. Reproduction, Nutrition, Developpement 22 1035–1047. (https://doi.org/10.1051/rnd:19820714)Search Google Scholar
Export Citation
Fabbri R, Vicenti R, Macciocca M, Pasquinelli G, Lima M, Parazza I, Magnani V & Venturoli S 2012Cryopreservation of ovarian tissue in pediatric patients. Obstetrics and Gynecology International 2012910698. (https://doi.org/10.1155/2012/910698)Search Google Scholar
Export Citation
Faire M, Skillern A, Arora R, Nguyen DH, Wang J, Chamberlain C, German MS, Fung JC & Laird DJ2015 Follicle dynamics and global organization in the intact mouse ovary. Developmental Biology 40369–79. (https://doi.org/10.1016/j.ydbio.2015.04.006)Search Google Scholar
Export Citation
Fransolet M, Labied S, Henry L, Masereel MC, Rozet E, Kirschvink N, Nisolle M & Munaut C 2014Strategies for using the sheep ovarian cortex as a model in reproductive medicine. PLoS ONE 9e91073. (https://doi.org/10.1371/journal.pone.0091073)Search Google Scholar
Export Citation
Gastal EL 2011 Ovulation: Part 2. Ultrasonographic morphology of the preovulatory follicle. In Equine Reproduction, pp. 2020–2031. Eds McKinnon AO, Squires EL, Vaala WE, Varner DD. Ames, IA: Blackwell Publishing.Search Google Scholar
Export Citation
Gastal GDA, Alves BG, Alves KA, Paiva SO, de Tarso SGS, Ishak GM, Bashir ST & Gastal EL 2017aEffects of cryoprotectant agents on equine ovarian biopsy fragments in preparation for cryopreservation. Journal of Equine Veterinary Science 53 86–93. (https://doi.org/10.1016/j.jevs.2016.03.014)Search Google Scholar
Export Citation
Gastal GDA, Hamilton A, Alves BG, de Tarso SGS, Feugang JM, Banz WJ, Apgar GA, Nielsen CK & Gastal EL 2017b Ovarian features in white-tailed deer (Odocoileus virginianus) fawns and does. PLoS ONE 12 e0177357. (https://doi.org/10.1371/journal.pone.0177357)Search Google Scholar
Export Citation
Gastal EL, Aguiar FLN, Gastal GDA, Alves KA, Alves BG & Figueiredo JR 2020 Harvesting, processing, and evaluation of in vitro-manipulated equine preantral follicles: a review. Theriogenology156 283–295. (https://doi.org/10.1016/j.theriogenology.2020.06.044)Search Google Scholar
Export Citation
Gaytan F, Morales C, Leon S, Garcia-Galiano D, Roa J & Tena-Sempere M 2015 Crowding and follicular fate: spatial determinants of follicular reserve and activation of follicular growth in the mammalian ovary. PLoS ONE 10 e0144099. (https://doi.org/10.1371/journal.pone.0144099)Search Google Scholar
Export Citation
Ginther OJ 2012 The mare: a 1000-pound guinea pig for study of the ovulatory follicular wave in women. Theriogenology 77 818–828. (https://doi.org/10.1016/j.theriogenology.2011.09.025)Search Google Scholar
Export Citation
Ginther OJ, Gastal MO, Gastal EL, Jacob JC, Siddiqui MAR & Beg MA 2008 Effects of age on follicle and hormone dynamics during the oestrus cycle in mares. Reproduction, Fertility and Development 20955–963. (https://doi.org/10.1071/RD08121)Search Google Scholar
Export Citation
Ginther OJ, Gastal MO, Gastal EL, Jacob JC & Beg MA 2009 Age-related dynamics of follicles and hormones during an induced ovulatory follicular wave in mares. Theriogenology 71 780–788. (https://doi.org/10.1016/j.theriogenology.2008.09.051)Search Google Scholar
Export Citation
Gleicher N & Barad DH 2011 Dehydroepiandrosterone (DHEA) supplementation in diminished ovarian reserve (DOR). Reproductive Biology and Endocrinology 9 67. (https://doi.org/10.1186/1477-7827-9-67)Search Google Scholar
Export Citation
Goncalves GR, Morotti F, Colombo AHB, Bonato DV, Bizarro-Silva C, Rosa CO, Cavalieri FLB & Seneda MM 2020 Influence of age and ovarian antral follicle count on the reproductive characteristics of embryo donor mares. Veterinary Record 186 564. (https://doi.org/10.1136/vr.105526)Search Google Scholar
Export Citation
Gonzales SM, da Silva CB, Lindquist AG, Bufalo I, Morotti F, Lisboa LA & Seneda M 2017 Regional distribution and integrity of equine ovarian pre-antral follicles. Reproduction in Domestic Animals 52836–841. (https://doi.org/10.1111/rda.12986)Search Google Scholar
Export Citation
Gougeon A & Chainy GBN 1987 Morphometric studies of small follicles in ovaries of women at different ages. Journal of Reproduction and Fertility 81 433–442. (https://doi.org/10.1530/jrf.0.0810433)Search Google Scholar
Export Citation
Haag KT, Magalhães-Padilha DM, Fonseca GR, Wischral A, Gastal MO, King SS, Jones KL, Figueiredo JR & Gastal EL 2013 Quantification, morphology, and viability of equine preantral follicles obtained via the Biopsy Pick-Up method. Theriogenology 79 599–609. (https://doi.org/10.1016/j.theriogenology.2012.11.012)Search Google Scholar
Export Citation
Kezele P, Nilsson E & Skinner MK 2002 Cell-cell interactions in primordial follicle assembly and development. Frontiers in Bioscience 7 d1990–d1996.Search Google Scholar
Export Citation
Kimura J, Kakusho N, Yamazawa K, Hirano Y, Nambo Y, Yokota H & Himeno R 2009Stereolithographic biomodelling of equine ovary based on 3D serial digitizing device. Journal of Veterinary Science 10 161–16 3. (https://doi.org/10.4142/jvs.2009.10.2.161)Search Google Scholar
Export Citation
Kristensen SG, Rasmussen A, Byskov AG & Andersen CY 2011 Isolation of pre-antral follicles from human ovarian medulla tissue. Human Reproduction 26 157–166. (https://doi.org/10.1093/humrep/deq318)Search Google Scholar
Export Citation
Lopes KRF, Lima GL, Bezerra LGP, Barreto-Junior RA, Oliveira MF & Silva AR 2017 Characterization of the ovarian preantral follicle populations and its correlation with age and nutritional status in Brazilian Northeastern donkeys (Equus asinus). Animal Reproduction Science 187 193–202. (https://doi.org/10.1016/j.anireprosci.2017.11.007)Search Google Scholar
Export Citation
Lucci CM, Amorim CA, Rodrigues APR, Figueiredo JR, Báo SN, Silva JRV & Gonçalves PBD 1999Study of preantral follicle population in situ and after mechanical isolation from caprine ovaries at different reproductive stages. Animal Reproduction Science 56 223–236. (https://doi.org/10.1016/s0378-4320(9900045-7)Search Google Scholar
Export Citation
Lucci CM, Rumpf R, Figueiredo JR & Báo SN 2002 Zebu (Bos indicus) ovarian preantral follicles: morphological characterization and development of an efficient isolation method. Theriogenology 571467–1483. (https://doi.org/10.1016/s0093-691x(0200641-6)Search Google Scholar
Export Citation
Malhi PS, Adams GP & Singh J 2005 Bovine model for the study of reproductive aging in women: follicular, luteal, and endocrine characteristics. Biology of Reproduction 73 45–53. (https://doi.org/10.1095/biolreprod.104.038745)Search Google Scholar
Export Citation
Malki S, Tharp ME & Bortvin AA 2015 A whole-mount approach for accurate quantitative and spatial assessment of fetal oocyte dynamics in mice. Biology of Reproduction 93 113. (https://doi.org/10.1095/biolreprod.115.132118)Search Google Scholar
Export Citation
Mihm M & Evans ACO 2008 Mechanisms for dominant follicle selection in monovulatory species: a comparison of morphological, endocrine and intraovarian events in cows, mares and women. Reproduction in Domestic Animals 43 (Supplement 2) 48–56. (https://doi.org/10.1111/j.1439-0531.2008.01142.x)Search Google Scholar
Export Citation
Nichols SM, Bavister BD, Brenner CA, Didier PJ, Harrison RM & Kubisch HM 2005 Ovarian senescence in the rhesus monkey (Macaca mulatta). Human Reproduction 20 79–83. (https://doi.org/10.1093/humrep/deh576)Search Google Scholar
Export Citation
Riley SC, Gibson AH, Leask R, Mauchline DJ, Pedersen HG & Watson ED 2001 Secretion of matrix metalloproteinases 2 and 9 and tissue inhibitor of metalloproteinases into follicular fluid during follicle development in equine ovaries. Reproduction 121 553–560. (https://doi.org/10.1530/rep.0.1210553)Search Google Scholar
Export Citation
Schmidt KL, Byskov AG, Nyboe Andersen A, Müller J & Yding Andersen C 2003 Density and distribution of primordial follicles in single pieces of cortex from 21 patients and in individual pieces of cortex from three entire human ovaries. Human Reproduction 18 1158–1164. (https://doi.org/10.1093/humrep/deg246)Search Google Scholar
Export Citation
Silva-Santos KC, Santos GM, Siloto LS, Hertel MF, Andrade ER, Rubin MI, Sturion L, Melo-Sterza FA& Seneda MM 2011 Estimate of the population of preantral follicles in the ovaries of Bos taurus indicusand Bos taurus taurus cattle. Theriogenology 76 1051–1057. (https://doi.org/10.1016/j.theriogenology.2011.05.008)Search Google Scholar
Export Citation
Tatone C, Amicarelli F, Carbone MC, Monteleone P, Caserta D, Marci R, Artini PG, Piomboni P & Focarelli R 2008 Cellular and molecular aspects of ovarian follicle ageing. Human Reproduction Update 14 131–142. (https://doi.org/10.1093/humupd/dmm048)Search Google Scholar
Export Citation
Woodruff TK & Shea LD 2011 A new hypothesis regarding ovarian follicle development: ovarian rigidity as a regulator of selection and health. Journal of Assisted Reproduction and Genetics 28 3–6. (https://doi.org/10.1007/s10815-010-9478-4)Search Google Scholar
Export Citation

Figure 1
Illustration of experimental procedures performed to assess preantral follicle morphology, classification, spatial distribution, density, and population in the equine ovary. Ovaries were divided into (A) three portions (lateral, n = 2; intermediary, n = 1), followed by (B) histological processing and sectioning to allow for evaluation of follicle density and population. The whole follicle population was estimated per ovary using a formula, as previously described (Gougeon & Chainy 1987, Gastal et al.2017b). (C, D, and E) A square grid sheet with columns and rows represented by letters and numbers, respectively, was placed upon the histological slides for microscopic evaluation. (D) Geometric center was determined for each histological section. (E) Analysis of preantral follicle distribution (distance in mm and angle in relation to the geometric center) and determination of dorsal and ventral regions of the ovary; five representative histological sections per ovarian portion per mare, now termed ‘ovarian maps’ (n = 5 maps per portion; 15 maps per ovary; 240 maps total for all 16 ovaries from 8 mares), were made. Representative histological sections depicting (F and G) lower and (I and J) higher follicle (primordial, transitional, and primary) density per microscopic field are shown in low magnification. (H) Normal and (K) abnormal late secondary follicles are shown in high magnification. (F, G, I, and J) Scale bars = 100 µm, magnification = ×200; and (H and K) scale bars = 50 µm, magnification = ×400. (A–E) Diagrams adapted from Alves et al. (2018).
Citation: Reproduction and Fertility 3, 2; 10.1530/RAF-21-0100Download Figure
Download figure as PowerPoint slide

https://raf.bioscientifica.com/view/journals/raf/3/2/RAF-21-0100.xml
We recommendOvarian fragment sizes affect viability and morphology of preantral follicles during storage at 4°C
G D A Gastal et al.,Point of view, 2017
Radiation-induced ovarian follicle loss occurs without overt stromal changes
Bruce F Kimler et al.,Reproduction, 2018
Ovarian fragment sizes affect viability and morphology of preantral follicles during storage at 4°C
G D A Gastal et al.,Reproduction, 2017
Vascular supply as a discriminating factor for pig preantral follicle selection
A Martelli et al., Point of view, 2009
Transforming growth factor-β: its role in ovarian follicle development
Davina Rosairo et al., Reproduction, 2008
Influence of age and ovarian antral follicle count on the reproductive characteristics of embryo donor mares
Gustavo Romero Goncalves et al.,Vet Record, 2020
Morphological features of the ovaries during oogenesis of the Oriental fruit fly, Bactrocera dorsalis, in relation to the physiological state
Ming-Yi Chou et al.,Journal of Insect Science
The effect of dietary protein on reproduction in the mare. III. Ovarian and uterine changes during the anovulatory, transitional and ovulatory periods in the non-pregnant mare
F.E. Van Niekerk et al., Journal of the South African Veterinary Association, 1997
Functional response of systemic and intrafollicular placental growth factor in cycling mares
Katiuska Satué et al., Acta Veterinaria Hungarica, 2020
Oocyte-Specific Deletion of Pten Causes Premature Activation of the Primordial Follicle Pool
Pradeep Reddy et al., Science, 2008
Equine granulosa cell tumours among other ovarian conditions: Diagnostic challenges
Catherine D. Renaudin, Audrey A. Kelleman, Kevin Keel, Jaye L. McCracken, Barry A. Ball, Ryan A. Ferris, Patrick M. McCue, Ghislaine Dujovne, Alan J. Conley
First published: 11 May 2020
https://doi.org/10.1111/evj.13279
Citations: 5
Funding information
John P. Hughes Endowment of the University of California-Davis and the Albert G. Clay Endowment of the University of Kentucky.
The peer review history for this article is available at https://publons.com/publon/10.1111/evj.13279
https://beva.onlinelibrary.wiley.com/doi/10.1111/evj.13279
The anatomy of the horse, a dissection guide. Horses. 224 THE ANATOMY OF THE HOBSB. but for the greater part of its extent the partition is composed of a plate of cartilage—the septal cartilage. This septal cartilage is continuous above with the perpendicular plate, which is merely an ossified portion of it; behind it is received into the cleft of the vomer, and expands on the premaxillary suture ; in front it expands on the inner aspect of the internasal suture; and inferiorly the alar cartilages are movably con- nected to it. The Inferior Extremities of the nasal fossee are termed the infe
Captions are provided by our contributors.
RMImage ID:RE3HHX
Preview
Save
Share
IMAGE DETAILS
Contributor:The Book Worm / Alamy Stock Photo
File size:7.1 MB (566.9 KB Compressed download)
Releases:Model - no | Property - noDo I need a release?
Dimensions:1715 x 1457 px | 29 x 24.7 cm | 11.4 x 9.7 inches | 150dpi
More information:
This image is a public domain image, which means either that copyright has expired in the image or the copyright holder has waived their copyright. Alamy charges you a fee for access to the high resolution copy of the image.
This image could have imperfections as it’s either historical or reportage.
. The anatomy of the horse, a dissection guide. Horses. 224 THE ANATOMY OF THE HOBSB. but for the greater part of its extent the partition is composed of a plate of cartilage—the septal cartilage. This septal cartilage is continuous above with the perpendicular plate, which is merely an ossified portion of it; behind it is received into the cleft of the vomer, and expands on the premaxillary suture ; in front it expands on the inner aspect of the internasal suture; and inferiorly the alar cartilages are movably con- nected to it. The Inferior Extremities of the nasal fossee are termed the inferior. Fig. 28. Transverse Section through the Nasal ChambJ'^rs. 1, Anterior tvirbinated bone ; 2. Posterior turbinated bone; 3. Anterior meatus; 4. Middle meatus; 5. Posterior meatus ; C. Septum nasi. nares, or, in common language, the nostrils. They have already been described (page 180). The Superior Extremities are separated from the cranial cavity by the cribriform plate of the ethmoid bone, and are occupied by the lateral masses of the same bone. Below and behind these are the superior nares—the large patent orifices by which the nasal fosste communicate with the pharynx, the right and left openings being separated by the vomer bone. The following openings into the nasal fossa should be found:— 1. The Opening of the Lachrymal Duct (ductus ad nas>im).—Look for this on the floor of the nasal fossa, a few inches within the nostril. It is easily seen in the living animal, and has already been referred to in. Please note that these images are extracted from scanned page images that may have been digitally enhanced for readability - coloration and appearance of these illustrations may not perfectly resemble the original work.. McFadyean, John, Sir, 1853-. Edinburgh, W. & A. K. Johnston, Limited; New York, W. R. Jenkins Co.Show morehttps://www.alamy.com/the-anatomy-of-the-horse-a-dissection-guide-horses-224-the-anatomy-of-the-hobsb-but-for-the-greater-part-of-its-extent-the-partition-is-composed-of-a-plate-of-cartilagethe-septal-cartilage-this-septal-cartilage-is-continuous-above-with-the-perpendicular-plate-which-is-merely-an-ossified-portion-of-it-behind-it-is-received-into-the-cleft-of-the-vomer-and-expands-on-the-premaxillary-suture-in-front-it-expands-on-the-inner-aspect-of-the-internasal-suture-and-inferiorly-the-alar-cartilages-are-movably-con-nected-to-it-the-inferior-extremities-of-the-nasal-fossee-are-termed-the-infe-image232419654.html
March 20, 2015
Breeding
The weather is getting warmer, the stallion has been chosen, the contract has been signed, and you think you may have seen your mare looking a little too interested in the gelding across the fence – time to call the clinic and set up a date to drop her off for breeding!
Whether this is your first adventure into the world of mares and foals, or you have been down this road before, you may be wondering what happens between the time you say your farewells at the clinic and the time you get that wonderful news. Well, wonder no more! In this article I will explain all of the techniques, technologies, and tricks we have at our disposal to make the process as reliable and smooth as possible. Keep in mind that every mare is very different and so the management approaches are specifically tailored to each individual case.
Before we delve into this fascinating topic, let’s review the typical reproductive cycle of the mare. Horses are what we call “long day breeders”, which means their breeding season happens when the days are getting longer during the springtime, roughly April to August. This is entirely related to light-dark cycles. During the winter, the mare is in anestrous, a state during which very little is happening in her reproductive tract. She does not cycle or come into heat during this time. You may have heard of placing a mare “under lights” – this tricks her brain into thinking that the days are getting longer, therefore bringing her into an earlier heat.
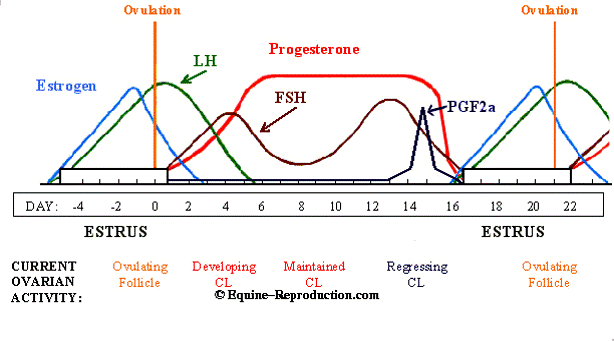
Diagram of the hormone profile during a regular reproductive cycle in the mare
The typical reproductive cycle lasts 21 days, and consists of two phases – the estrous phase (in heat) and the diestrous phase (out of heat).
The anatomy of the mare’s reproductive tract is quite similar to that of many other species. The uterus is divided into the body and two uterine horns. Each horn leads to the oviduct and then the infundibulum, ending at the ovary.
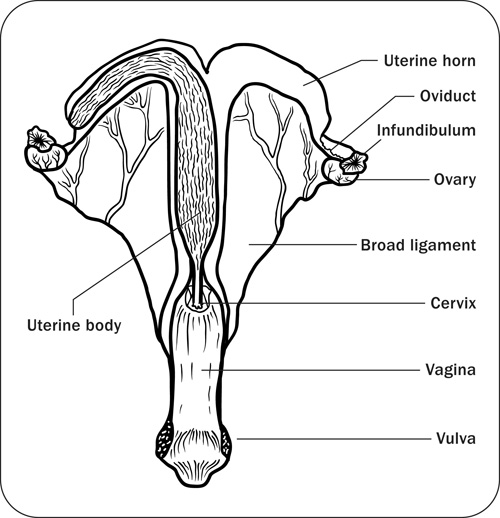
Representation of the mare reproductive tract
The typical reproductive cycle lasts 21 days, and consists of two phases – the estrous phase (in heat) and the diestrous phase (out of heat).
The anatomy of the mare’s reproductive tract is quite similar to that of many other species. The uterus is divided into the body and two uterine horns. Each horn leads to the oviduct and then the infundibulum, ending at the ovary.
The broad ligament carries the blood supply to the reproductive tract, and suspends the tract in place.
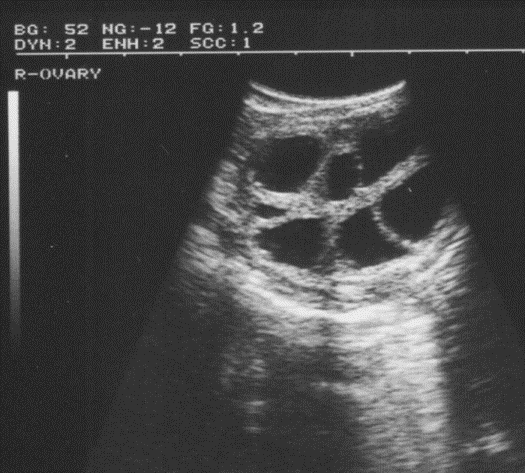
Ultrasound Image of ovary with multiple follicles (dark areas) present
The ovary, when active, is always changing. The main two structures you will find on the ovary are the follicle and the corpus luteum, or the CL. These two structures are extremely important in reproductive management.
The follicle produces estrogen, the hormone responsible for behavioural estrous in the mare. The follicle also contains the ovum. The ovum is released from the follicle during ovulation, to become fertilized by the spermatocyte.
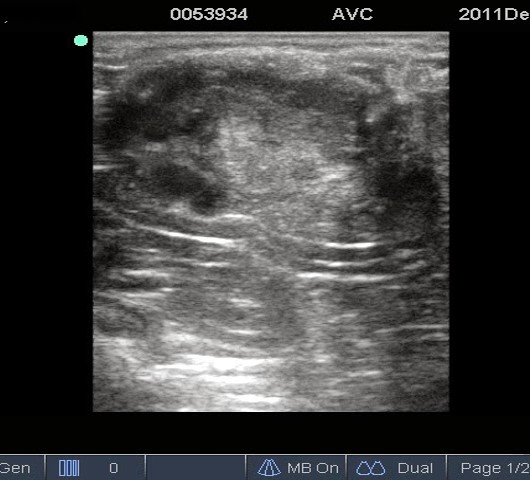
Ultrasound image of an ovary with a corpus luteum (CL) present (Solid structure in centre of ovary)
The CL is what develops from the old follicle once it has ovulated. The CL produces progesterone, the hormone responsible for suppression of behavioural estrous and maintenance of pregnancy. It regresses after approximately 14 days, which will allow for the development of another follicle, and the mare will again display signs of behavioural estrous.
Now that we have all of that anatomical and physiological stuff out of the way, we can talk about what we actually do with that information!
Our main tools for monitoring your mare are a digital ultrasound machine and palpation. We perform periodic ultrasounds and palpations via the rectum to view and feel the whole reproductive tract, especially the ovaries. By palpating and viewing the follicles, we are able to follow their growth and eventual ovulation. Several signs, including the tone or softness of the uterus, the sensitivity of the ovary, the follicle size/shape, and the uterine edema score, can provide us with an estimate of when the mare will ovulate.
When the mare is getting close to ovulation, she is then induced by administering a small amount of synthetic hormone. This allows us to predict the timing of ovulation much more accurately. This is particularly helpful for mares that will be bred with cooled semen being shipped from another province or from the USA, or for mares where only a few doses of frozen semen are available. By being able to predict when a mare will ovulate, we can use less semen than we would otherwise. Of course, not every mare remembers to check the clock before she ovulates, and so after administering the hormone we will check the mare more frequently to ensure that her ovulation is detected and she is inseminated as closely to ovulation as possible, which will help maximize our chances at getting a pregnancy.
Remember that pesky CL that produces progesterone and prevents your mare from coming back into heat? If the mare presents to the clinic with a CL on one of her ovaries, or if she unfortunately did not become pregnant from the last insemination, we are able to cause early regression or “lysis” of the CL by administering a hormone called prostaglandin specifically designed for this purpose. Once the prostaglandin is administered, the CL will regress and the mare will come back into heat in the next 3-7 days, depending on the size of the next biggest follicle on her ovaries. This process is called “short-cycling”.
There are several other management strategies that we sometimes must employ to help our less fertile mares.
Uterine Lavage – to do this, we aseptically introduce a special catheter into the uterus and flush the uterus with saline or a similar liquid. The amount of fluid varies between mares, depending on our assessment of the fluid that we receive back. Mares that require this procedure usually have problems clearing excess fluid from the uterus following breeding, or tend to accumulate more fluid to begin with. To make the uterus a healthy environment for the embryo, it is important to help the mare evacuate all excess fluid if she is unable to do so on her own.
Oxytocin administration – many mares that have trouble clearing fluid may simply not have enough mechanisms to help their uterus to contract. Oxytocin is the same endogenous hormone that is released during nursing and helps to clear the placenta and bring the uterus down to normal size after foaling. By administering oxytocin we help the mare to do the job of making the uterus a healthy environment.
Uterine Infusions – this involves infusing an antibiotic or another drug into the uterus. This strategy is only typically used in special cases when we have diagnosed a uterine infection.
Synthetic Progesterone – we will sometimes supplement a mare with a synthetic progesterone analog that will help the mare maintain her pregnancy or reset her cycle. If your mare has had problems with early embryonic loss in the past, we may suggest starting her on this supplement.
Finally, no article on mare reproduction is complete without discussing embryo transfer. Embryo transfer (ET) involves removing a fertilized embryo from one mare (the “donor”), and placing it into the uterus of another mare (the “recipient”). It is very important that the donor and recipient mares’ cycles are synchronized, so that when the embryo is placed into the recipient’s uterus, she does not reject it. Synchronizing their cycles involves inducing the recipient to ovulate about 1 day after the donor – this timing carries the best results for pregnancy. Most of the hormones and prostaglandins mentioned earlier are used to help synchronize these mare’s cycles, in addition to a few others. The advantages of ET are that a mare can mother more than one foal in a year (depending on the breed association), and mares that are at risk or may have trouble carrying a foal to term can still mother foals. Another advantage is that competition mares are then able to take less time off from their busy schedules.
Once your mare is bred, it is time to wait for the magic to happen! Using the ultrasound machine, we are able to detect signs of pregnancy as early as 14 days after ovulation. At this point, we are unable to see an embryo, but can only see the round, fluid-filled vesicle in which the embryo resides. Once the vesicle is seen at 14 days, the pregnancy is then confirmed at 25 days post-ovulation, when the embryo and heartbeat are visible.
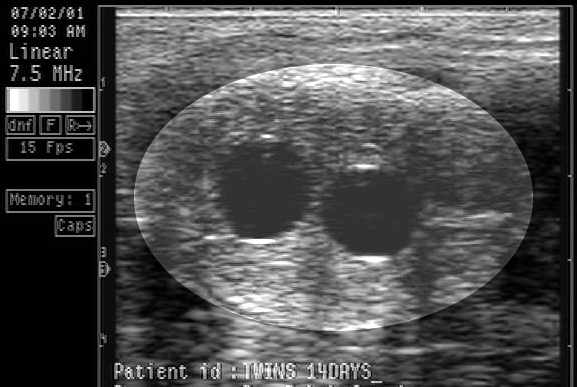
Ultrasound image of two vesicles present in within the uterus (consistent with twins)
Twins. They seem like such a great problem to have, but unfortunately healthy twins are very rare and often do not survive at all. They can also carry a significant risk to the dam, which can compromise her future fertility, and sometimes, her life. Fortunately, if detected early enough, twins can be treated and avoided, leaving just one pregnancy behind to continue. Twins can usually be detected at the first pregnancy check, which is 14 days post-ovulation. They are not always treated at that time, but may require a second visit. Mares that have had twins in the past have a much higher risk of conceiving twins. Thoroughbreds statistically have a higher incidence of twinning.
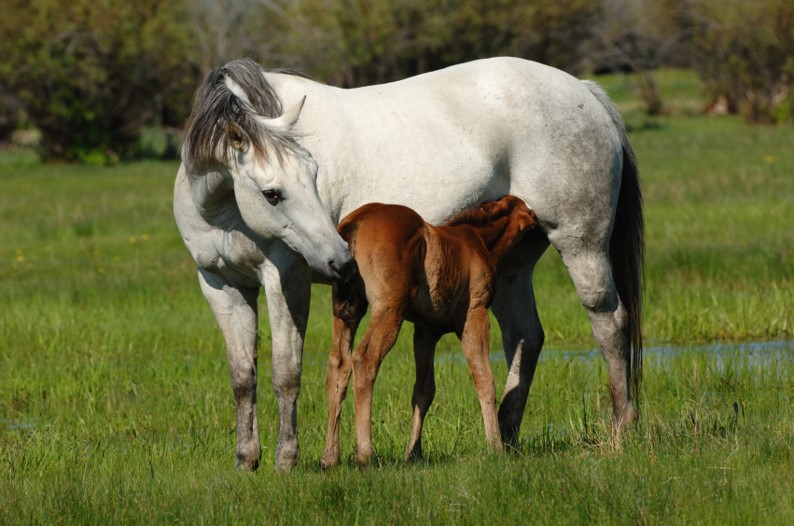
All of this may seem like a lot of work just to breed a mare, but these methods and technologies can really enhance your breeding operation, no matter how big or small. So the next time you drop off your mare at the clinic, rest assured that we are using the safest and most reliable means by which to make her a mother.. you will be hearing the clip-clop of tiny hooves in no time!
https://www.burwashequine.ca/blog/2015/3/20/breeding-your-mare-a-behind-the-scenes-look-at-the-science-of-mare-reproduction
Reproductive Tract Anatomy and Physiology of the Cow
Guide B-212
Reviewed by Jason Turner
College of Agricultural, Consumer and Environmental Sciences
Author: Professor/Extension Horse Specialist, Department of Extension Animal Sciences and Natural Resources, New Mexico State University (Print-friendly PDF)
Introduction
Understanding the anatomy and physiology of the cow's reproductive system is fundamental to good cattle management. Basic knowledge in this area will help producers do a better job of getting cows rebred, especially when using artificial insemination and estrus synchronization. It will also enable producers to better understand and control reproductive diseases and calving problems.
Anatomy
The ovary is the primary female reproductive organ and has two important functions: producing the female reproductive cell (the egg or ovum) and producing the hormones estrogen and progesterone. The cow's two ovaries are oval to bean-shaped organs that are 1—1.5 inches long and located in the abdominal cavity.
The secondary sex organs are a series of tubes that receive semen, transport sperm to the egg so it can be fertilized, nourish the fertilized egg (embryo), and allow the calf to be birthed. These organs include the vagina, cervix, uterus, uterine horns, and oviducts (also called Fallopian tubes), which each have a funnel-shaped opening called the infundibulum. Figure 1 presents a diagram of the complete reproductive tract anatomy.
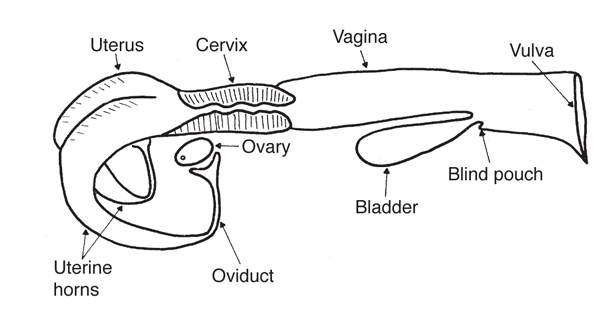
Figure 1. Diagram of the reproductive tract of the cow. (Adapted from Rich and Turman, n.d.)
The ovary produces the egg by a process called oogenesis. In contrast to the continuous production of sperm (spermatogenesis) in the male, oogenesis is cyclic. This cycle (called the estrous cycle) has a characteristic length and consists of a definite sequence of events, both physiological and behavioral.
The ovary contains several thousand tiny structures called primary follicles. Each follicle consists of a germ cell surrounded by a layer of cells. This germ cell has the potential to mature into an egg if the follicle completes development. However, most of the primary follicles never develop. Rather, they die, are absorbed by the ovary, and are replaced by newly formed primary follicles.
The relatively few primary follicles that develop completely do so through a series of phases. Many layers of cells are added to the single layer of cells surrounding the egg in the primary follicle, forming a central cavity. As the follicle and cavity grow larger, the egg becomes attached to the back of the follicle (opposite the ovulation site) by a stalk of cells. As the follicle continues to grow rapidly, the side opposite the egg bulges from the surface of the ovary and becomes very thin. Once the follicle reaches this mature state it is called a Graafian follicle. At ovulation, the thin portion ruptures to release the contents of the follicle, including the egg.
Following ovulation, the cells that developed within the follicle differentiate to form the corpus luteum, which has the important function of producing progesterone.
The released egg is caught by the infundibulum and moves into the oviduct, where fertilization occurs if viable sperm are present. The egg remains capable of fertilization for only a few hours. Thus, it is very important that fertile sperm be present near the time of ovulation. The egg moves through the oviduct and into the uterus within the next three to four days. If it is fertilized, it begins embryological development in the uterus. If it is not fertilized, it degenerates.
The body of the uterus is relatively short and poorly developed, while the uterine horns are longer and well developed. The embryo develops in the uterine horns. The fetus develops within a layer of membranes called the placenta. The mother nourishes the fetus via diffusion of nutrients across the placenta. There is no direct blood connection between fetus and mother.
The cervix is essentially the neck of the uterus. It has thick walls and a small opening that is difficult to penetrate because of overlapping or interlocking folds (annular rings). It serves as a passageway for sperm deposited in the vagina and for the fetus at the time of birth. During pregnancy, it is usually filled with a thick secretion that serves as a plug to protect the uterus from infective material entering through the vagina.
The vagina serves as the receptacle for the male penis during copulation. During natural mating, semen is deposited in the vagina near the cervix. The urinary bladder opens to the exterior through the urethra, which opens into the vagina. This region of the vagina is restricted in size because of sphincter muscles associated with the urethral opening. The region behind the external urethral orifice is called the vestibule and is a common passageway for both the urinary and the reproductive systems. The external opening of the vagina is called the vulva.
Hormonal Regulation of the Female Reproductive Tract
Normal reproduction in the female depends on hormones, which are specific chemical substances produced by specialized glands called endocrine glands. These secretions pass into the blood and lymph fluids and are transported to various parts of the body, where they exert several specific effects. Reproductive hormones may originate in the hypothalamus, pituitary, gonads, uterus, or placenta.
Hypothalamic hormones are produced in the hypothalamus, a portion of the brain that is the neural control center for reproductive hormones. The role of hypothalamic hormones is to regulate the release of other hormones from the pituitary gland, and many hypothalamic hormones are therefore called releasing hormones. The primary releasing hormone of reproduction is gonadotropin-releasing hormone (GnRH). GnRH controls the release of follicle-stimulating hormone (FSH) and luteinizing hormone (LH) from the pituitary gland, located at the base of the brain. These pituitary hormones regulate the production of estrogen and progesterone from the ovary. FSH stimulates the follicle's growth, development, and function, while LH causes the follicle to rupture and the corpus luteum to develop.
The female hormone estrogen is produced by the Graafian follicle on the ovary. Another hormone originating from the ovary is progesterone, which is produced by the corpus luteum. Each has an important role in the female reproductive process.
Estrogen regulates several functions: the development and functioning of the secondary sex organs; the onset of heat, or estrus (the period of sexual receptivity); the rate and type of body tissue growth, especially fat deposition; and priming or preparing of the prepubertal heifer and postpartum cow for the onset of sexual activity.
Progesterone is the hormone of pregnancy. It suppresses the further development of follicles and estrogen secretion. While progesterone is being produced, the female does not exhibit estrus. Progesterone is necessary for preparing the uterus to receive the fertilized egg and maintains the proper uterine environment for pregnancy. Estrogen and progesterone are not completely separate in their effects because both are necessary for complete development of some important organs. Uterine development is initiated by estrogen and completed by progesterone. The fertilized egg will not attach and survive in the uterus unless that tissue has been properly prepared by the action of estrogen and progesterone. Estrogen causes rhythmic contractions of the uterus. On the other hand, progesterone has a quieting effect on the uterus, so there are no contractions that might disturb pregnancy.
Complete development of the mammary gland also depends on both hormones. Estrogen promotes the growth of the duct system, and progesterone is necessary for the development of the clusters of milk-secreting alveoli on the ducts. In general, estrogen makes things happen and progesterone calms them down.
A number of other hormones and hormone-like chemicals are known to have important roles in regulating the cow's reproductive system. Prostaglandins are secreted by many body tissues and perform a variety of functions. The prostaglandin primarily affecting the cow's estrous cycle is prostaglandin F2α (PGF2α), which is produced by the uterus. PGF2α is the natural luteolytic agent that causes the corpus luteum to regress late in the estrous cycle and allows a new estrous cycle to be initiated in the nonpregnant female. In a pregnant cow, a signal is sent from the developing embryo to the uterus to prevent PGF2α release, which allows the corpus luteum to persist throughout pregnancy. Maternal recognition of pregnancy is believed to occur between days 16 and 17 after fertilization. Injecting cows or heifers with PGF2α between days 6 and 16 of the estrous cycle will cause premature regression of the corpus luteum, with the best results achieved among females injected on days 10 to 16.
Injecting PGF2α during the first five (1—5) and last five (17—21) days of the estrous cycle will generally not cause luteal regression. The luteolytic response allows the use of PGF2α in estrus synchronization programs in cow herds and initiates abortion in feedlot heifers. Estrogens, progestins, prostaglandins, and GnRH have all been used in various combinations in effective estrus synchronization programs.
The Estrous Cycle
The cow's reproductive cycle consists of a series of events that occur in a definite order over a period of days. The estrous cycle in the cow averages 21 days (range is 17—24). During this time, the reproductive tract is prepared for estrus or heat (the period of sexual receptivity) and ovulation (egg release). Figures 2 and 3 outline the sequence of anatomical and hormonal changes that occur during a typical 21-day cycle in which pregnancy does not occur.
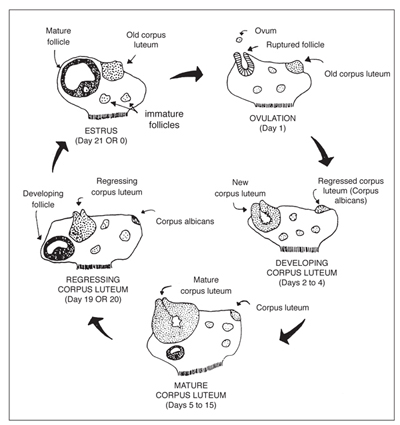
Figure 2. Diagram of a 21-day estrous cycle in which pregnancy does not occur. (Adapted from Deutscher, 1980)
Day 0: The cow is in estrus (standing heat) due to an increased concentration of estrogen. As estrogen levels reach a certain threshold level, a surge of LH is released by the pituitary. Near the end of standing heat, the mature Graafian follicle ovulates (ruptures) in response to this LH surge.
Days 1—2: The cells that formerly lined the follicle change and become the luteal cells of the corpus luteum. This change in cell form is caused by hormonal action, primarily the action of LH.
Days 2—5: The corpus luteum grows rapidly in both size and function. At this stage, numerous follicles may be seen on the ovary, but by day 5 they have begun to regress.
Days 5—16: The corpus luteum continues to develop and typically reaches its maximum growth and function by day 15 or 16. It secretes the hormone progesterone, which inhibits (blocks) LH release by the pituitary gland. During this period, the ovaries are relatively inactive except for the functional corpus luteum. No follicles reach maturity and/or ovulate because of high concentrations of progesterone.
Days 16—18: Increased follicular growth and accompanying estrogen secretion by the ovary stimulate PGF2αsecretion by the uterus, causing rapid regression of the corpus luteum.
Days 18—19: The corpus luteum is almost nonfunctional and progesterone release is suppressed, removing the blocking action of progesterone on LH and FSH. Of the several follicles that are initially recruited, one becomes dominant by a surge in rapid growth and activity. As this Graafian follicle grows, it secretes increasing amounts of estrogen, and the smaller follicles regress.
Days 19—20: With the increase in estrogen release by the Graafian follicle and a corresponding decrease in progesterone by the regressing corpus luteum, estrus or heat will occur (cycle has now returned to day 0). The high estrogen concentration in the blood triggers a release of LH near the onset of heat. Following this surge in LH blood concentrations, the mature follicle ruptures to release the egg, and the cellular tissue left behind becomes luteinized and forms a new corpus luteum (cycle has now returned to days 1—2). Progesterone again becomes the dominant hormone.
The timing given for these events is only approximate (based on a 21-day average) and differs for different cycle lengths (range of 17—24 days).
This discussion of events that occur during the estrous cycle is based on a full cycle in which pregnancy does not occur. If the egg is fertilized and begins developing in the uterus, the corpus luteum does not regress but continues to function and secrete progesterone. During pregnancy, no follicles develop to maturity and heat does not normally occur. Increased concentrations of progesterone promote uterine quiescence, providing the most favorable conditions for the developing fetus.
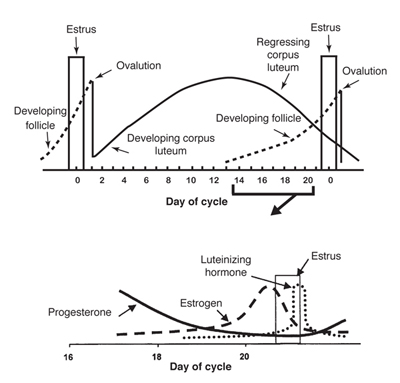
Figure 3. Graph of the anatomical and hormonal changes that occur during a typical 21-day estrous cycle. (Adapted from Deutscher, 1980)
Any condition that prolongs the period of time when blood concentrations of progesterone remain high (such as implanting, injecting, or feeding progestins for estrus synchronization) will keep the female from exhibiting estrus. Occasionally, the corpus luteum does not regress normally even though the animal does not become pregnant (pseudopregnancy).
Occasionally, abnormally short estrous cycles (7—11 days) occur. This condition appears to occur because either no corpus luteum is formed or, if one is formed, it is nonfunctional and progesterone concentration remains too low to sufficiently suppress the release of gonadotropins (FSH and/or LH) from the anterior pituitary.
Cows also have periods of anestrus (noncycling). For example, an anestrous period is commonly observed in cows following calving. Low levels of nutrition can contribute to the duration of anestrus, especially in young cows nursing calves.
Estrus is not always accompanied by ovulation, nor ovulation by estrus. Heat without ovulation (anovulatory heat) will not result in pregnancy, even if the female is bred. Ovulation without the external signs of heat (quiet or silent heats) is not uncommon in cows, especially the first few weeks after calving. Such females will generally not "stand" to be bred by a bull.
The discussion of the hormonal control of the estrous cycle and pregnancy as presented here is a greatly simplified summary of the whole process. In reality, the reproductive process is very complicated and involves a number of hormonal interactions and events.
References
Deutscher, G.H. 1980. Reproductive tract anatomy and physiology of the cow [G80-537-A]. Lincoln: University of Nebraska Cooperative Extension.
Rich, T.D., and E.J. Turman. n.d. Reproductive tract anatomy and physiology of the cow [Beef Cattle Handbook BCH-2200]. Stillwater: Oklahoma State University.
Original authors: Ron Parker and Clay Mathis, Extension Livestock Specialists. Adapted from Extension Beef Cattle Resource Committee Beef Cattle Handbook publication BCH-2200, by T.D. Rich and E.J. Turman, Animal Science Department, Oklahoma State University.
https://pubs.nmsu.edu/_b/B212/
https://www.cram.com/flashcards/anatomy-ii-female-reproduction-horse-1667046
Granulosa cell tumours in the mare: A review of 52 cases
C. E. Sherlock, K. Lott-Ellis, A. Bergren, J. M. Withers, D. Fews, T. S. Mair
First published: 12 September 2015
https://doi.org/10.1111/eve.12449
Citations: 12
Read the full text

TOOLS
SHARE
Summary
Granulosa cell tumour (GCT) is reported to be the most common ovarian neoplasm in the mare. A multicentre retrospective study evaluating the medical records of horses undergoing ovariectomy for treatment of a GCT was undertaken, documenting the presenting clinicopathological features, treatment and outcome. Follow-up information was obtained by telephone questionnaire. Fifty-two mares were included. Most presented with behavioural/reproductive abnormalities, including stallion-like behaviour (50%), aggression (31%), prolonged oestrus or nymphomania (19%), and persistent anoestrus (8%). Most (93%) affected ovaries had a multilocular appearance when examined ultrasonographically. Only 60% of horses with histopathologically confirmed GCTs demonstrated elevations in serum inhibin concentrations. Twenty-five percent of horses had histopathologically confirmed bilateral GCTs. Ninety-four percent of horses that underwent ovariectomy were discharged from the hospital. Standing laparoscopic ovariectomies had a lower complication rate (34%) than other surgical methods. Long-term complications were uncommon (11%). In conclusion, histopathologically confirmed GCTs can occur in the presence of normal serum inhibin concentrations. The high prevalence of bilateral GCTs indicates that thorough evaluation of the contralateral ovary is necessary. Surgical ovariectomy for treatment of GCTs has favourable short- and long-term outcomes. Standing laparoscopic ovariectomy may be associated with a lower complication rate than other surgical methods.https://beva.onlinelibrary.wiley.com/doi/10.1111/eve.12449
Equine AMH Testing
Introduction
Anti-Müllerian hormone (AMH) is a glycoprotein, important in the development of the reproductive tract in mammals including horses. Sertoli cells of the fetal testes produce AMH results in regression of the Müllerian ducts and development of Wolffian duct. Fetal ovaries do not produce AMH results in the development of Müllerian duct and regression of Wolffian duct. AMH concentration decreases in stallions after puberty. AMH plays an active role in folliculogenesis in mares after birth.
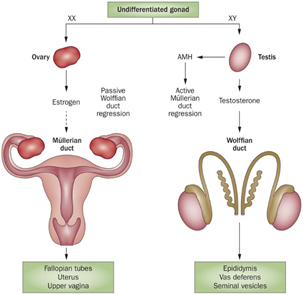
Clinical Applications
Cryptorchidism
Cryptorchidism is a condition in which one or both testes are retained within the inguinal canal and/or abdominal cavity. The circulating concentration of AMH in the cryptorchid stallion without scrotal testes is higher than intact stallion. But the levels of testosterone and estrogen are significantly low or not different between intact and cryptorchid stallions. Testicles are the only source of AMH, whereas testosterone secrets from testicular and adrenal glands.
Testicular Tissue, Function and Tumor
AMH is the biomarker to detect the retained testicular tissue in horses of all ages but testosterone is not useful in horses younger than 18 month of age, estrogen sulfate is less informative before 3 years of age. AMH concentration is higher in stallions during breeding season than non-breeding season. Compared to testosterone, serum AMH displays less diurnal variation making the measurement of AMH better for reproducibility. Measurement of serum AMH or immunohistochemistry can differentiate Sertoli cell tumors with other tumor types.
Ovarian Reserve and Function
AMH is synthesized from granulosa cells of the ovarian follicles in adult mares. Circulating concentration of AMH is used to differentiate ovariectomized and intact mares. The expression of AMH is strong in the small Antral follicles (15-20 mm) and faint signal in dominant and preovulatory follicles. AMH concentration varies between young, middle aged and old age mares. AMH is highly correlated to antral follicle count and the concentration is relatively stable during and between different estrous cycles therefore valuable in assessing the reproductive lifespan of mares.
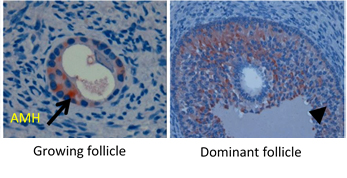
Granulosa Cell Tumor
The equine granulosa cell tumor (GCT), is the most common tumor of the equine ovary. In contrast with hemorrhagic anovulatory follicle or ovarian hematoma, the equine GCT is hormonally active. Inhibin and testosterone concentrations are increased in nearly 80% and 48% of GCT mares respectively. But their concentrations are also increased during pregnancy, and slightly increased testosterone concentrations in non-pregnant mares could be attributed to increased adrenal gland activity. Mares with a GCT have higher circulating AMH concentrations than non-pregnant and pregnant mares without a GCT indicating that AMH can serve as an endocrine marker for equine GCTs. AMH turns out to be the most important endocrine marker to identify mares with a GCT because it has a higher sensitivity (98%) than inhibin and testosterone.
Biomarker for Fertility in Mares
AMH and / or AFC has been associated with fertility in both assisted reproduction settings as well as natural fertility. Concentrations of AMH have been shown related to assisted reproduction parameters such as oocyte quality, response to ovarian stimulation protocols, embryo production and implantation in cattle. Studies have shown that in growing equine follicles, low AFC was associated with reduced expression of AMH, AMHRII, ESR2 and FSHR in granulosa cells compared to growing follicles from mares with high AFC. The AMH concentrations were higher in pregnant than in open mares based upon pregnancy outcome at Days 13-18.
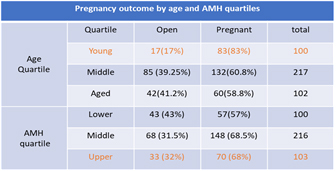
Serum AMH concentration were positively correlated with the daily increase in ovarian follicle diameter as well with mares with higher conception rates. It was also negatively correlated with anovulation and number of mating per conception. In summary, a strong relationship was found between peripheral AMH concentrations and fertility performance in mares.
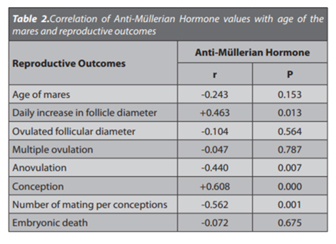
THE OVARY
Ovarian function and development
The ovaries function 1.) to produce ova, 2.) as an endocrine gland (i.e. secrete mediators into the bloodstream to act at a distant site). During ovarian development from the primordial gonad, structures termed sex cords form which are likely derived from mesothelial cells forming the gonads. These linear plates of tissue incorporate the migrating germ cells into their structure.
Oogenesis refers to the development of oocytes within the ovary. The process can be divided into three stages – fetal, prepuberty (resting stage) and puberty.
During fetal oogenesis, the sex cords break down and the germ cells undergo enhanced mitosis. In most mammals, this prolonged mitotic activity ceases before or soon after birth. These cells arrest in prophase of meiosis I (primary oocytes), are enclosed in basal lamina surrounded by follicular cells and are referred to as primordial follicles.
The following concepts must be understood regarding oogonia and oocytes in relationship to the prepubertal, pubertal and post pubertal periods.Primary oocytes enter a resting phase in which full maturation does not occur until stimulation by gonadotrophic hormones takes place (puberty).
Large numbers of post mitotic germ cells (oogonia) and primary oocytes undergo atresia or death in the prenatal period, the prepuberty period and the post puberty period.
Following puberty, the primary oocyte surrounded by follicular cells enters follicular maturation when stimulated by gonadotrophic hormones. This maturation occurs in cyclical waves. In regards to the developing follicle, the surrounding follicular cells proliferate, become stratified and change morphology from a spindle shaped cell to a cuboidal phenotype (called granulosa cells).
Completion of the first meiotic division results in the formation of the secondary oocyte and extrusion of the first polar body. This change typically takes place before ovulation, except in dogs, where formation of the secondary oocyte takes place after ovulation. The second meiotic division takes place during fertilization and results in the extrusion of the second polar body.
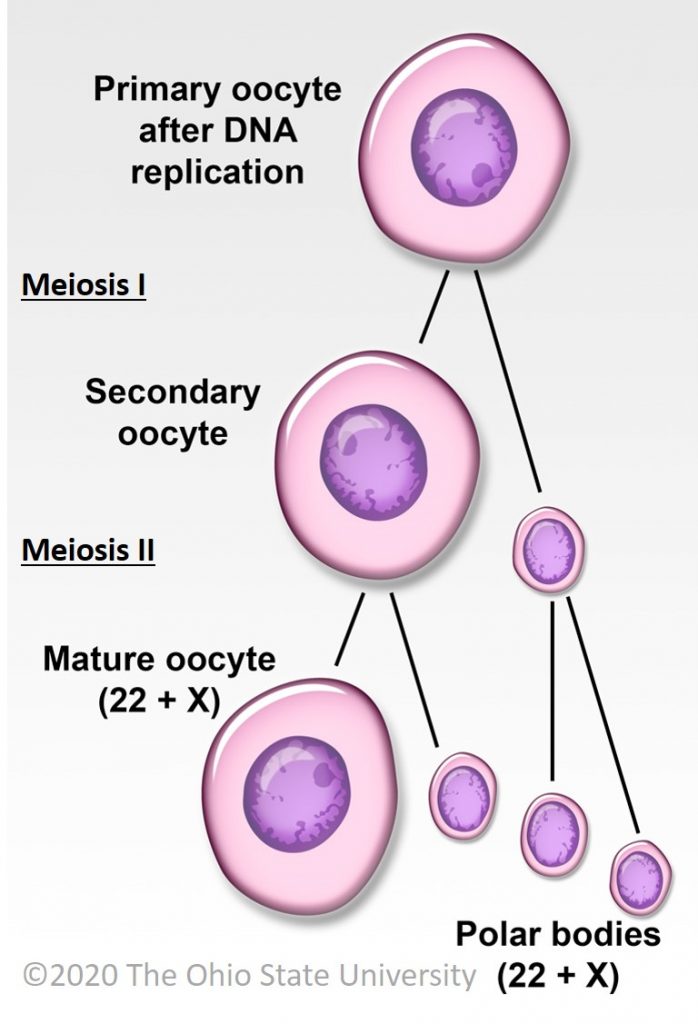
Ovarian structure and histologic organization
The ovary is divided anatomically into the cortex and medulla. The cortical aspect of the ovary is covered by cuboidal epithelium during development that converts to squamous epithelium with age. The cortical parenchyma is composed of follicles (oocytes and follicular cells), interstitial cells and collagenous connective tissue stroma. The ovarian medulla contains large arteries and veins, lymphatics, nerves embedded in a loose collagenous matrix. The rete ovarii are also present in the medulla, which are cords of cells found in the medulla homologous to the rete testis.
In most domestic species, the cortex contains the developing follicles. However in the horse, the anatomy of the ovary is reversed. In this species, the follicular development takes place in the region of the ovary corresponding to the medulla. A concavity forms in the ovary called the ovulation fossa, corresponding to the site in the equine ovary which ovulation occurs. In addition, ovaries in the equine fetus and neonates are markedly enlarged compared to the size of the animal. This enlargement in attributed primarily to hyperplasia and hypertrophy of the interstitial cells. Subsequent atrophy takes place post-natally.
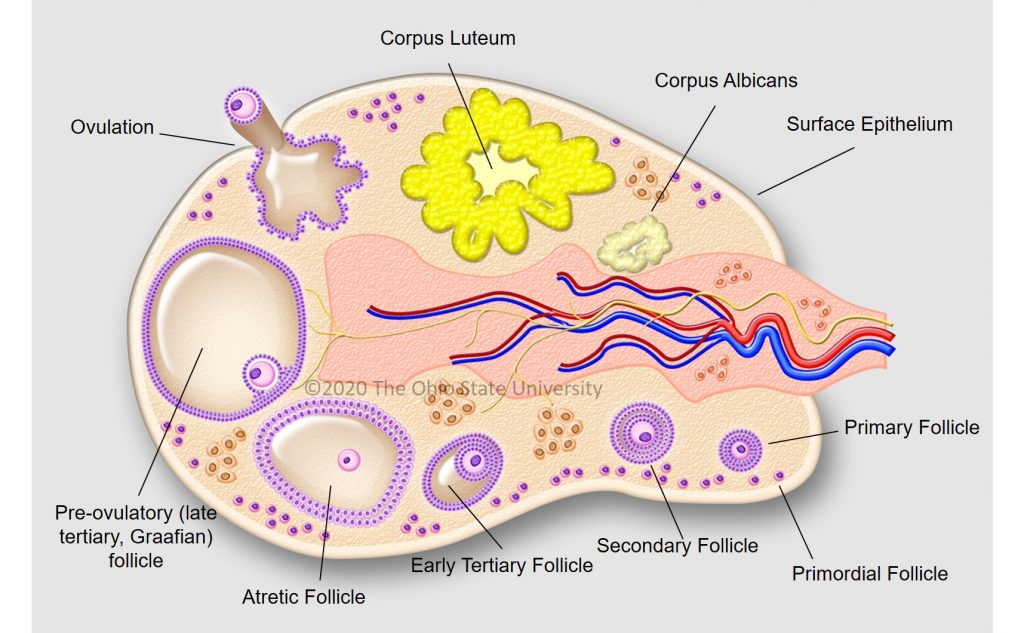
Follicular development
Development of the ovarian follicle is a sequential process which is primarily directed by the influence of gonadotrophins (follicle stimulating hormone [FSH] and luteinizing hormone [LH]) and can be variable between species.
The sequence of follicular development is 1.) primordial follicle, 2.) primary follicle, 3.) secondary follicle, 4.) tertiary follicles.Primordial follicles contain a primary oocyte and are surrounded by a single layer of flattened follicular cells.
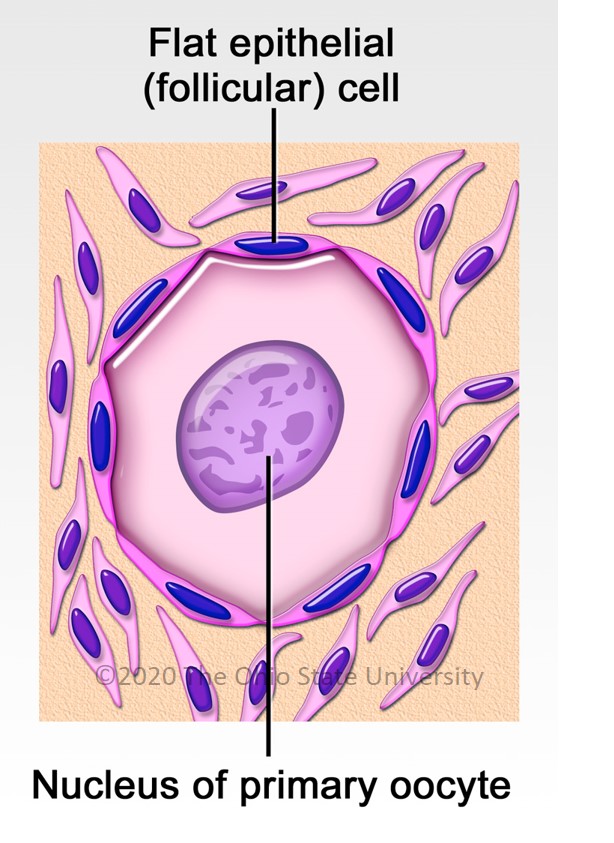
Primary follicles still contain a primary oocyte but the follicular cells become more cuboidal and are now known as granulosa cells. Follicular (granulosa) cells proliferate (membrane granulosa) but are separated from the oocyte by a thick periodic acid Schiff (PAS) positive basement membrane called the zona pellucida. The organized stromal cells around the follicles are called theca cells.
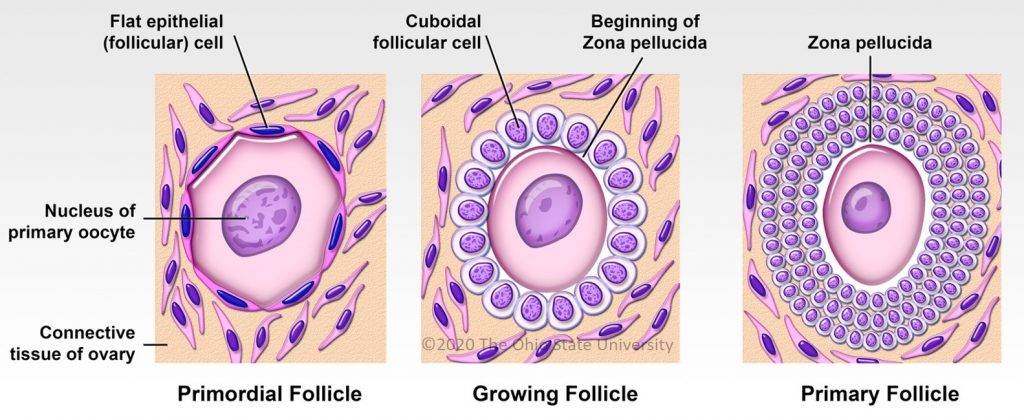
Secondary follicles start develop spaces between granulosa cells that coalesce to eventually form a large space called the follicular antrum. The granulosa cells secrete PAS positive material into these spaces. The stromal cells surrounding the follicle form two layers, the theca interna and the theca externa.
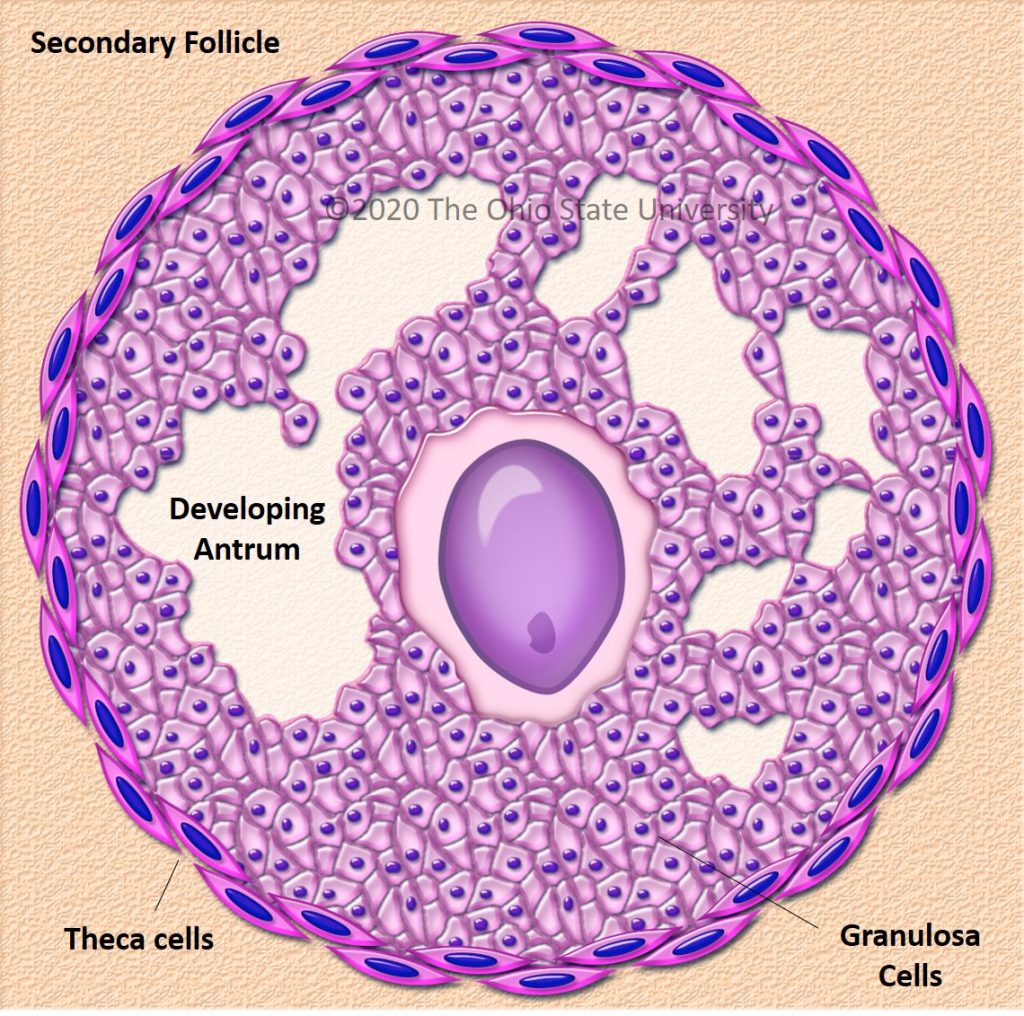
Graafian (tertiary, mature) follicles are large preovulatory follicles which bulge from the surface of the ovary. Once the follicular antrum is formed, the oocyte is surrounded by a remnant of granulosa cells called the cumulus oophorus. The cells of the cumulus oophorus immediately adjacent to the oocyte are known as the corona radiata.
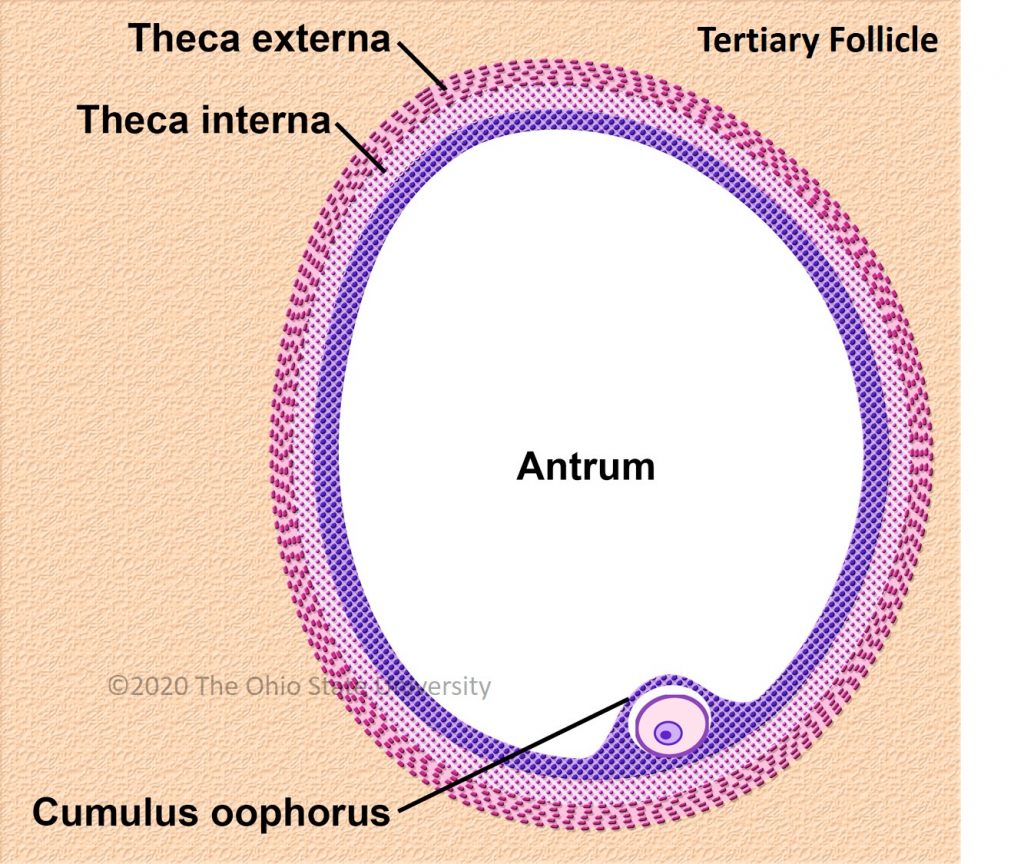
FIGURE(S): Follicles – Ovary
Endpoints of follicular development
A follicle only has two potential fates, atresia or ovulation. Follicular atresia or degeneration of the developing follicle can take place any time during development. During this process the oocyte liquefies with subsequent collapse of the zona pellucida and the wall of the follicle. Fibrous tissue, phagocytes and vascular tissue replace the region of the atretic follicle while the remnants of the theca interna undergo hypertrophy. Ovulation involves the rupture of the follicle and release of the oocyte. This is induced by a surge of luteinizing hormone released by the anterior pituitary. Only the corona radiata is present on the oocyte after release from the follicle.
Consequences of ovulation
The corpus hemorrhagicum is formed soon after ovulation from hemorrhage into the remnants of the follicular structure resulting in a large blood clot. The remaining granulosa cells and cells of the theca interna undergo hypertrophy, proliferate and invade the corpus hemorrhagicum, transforming into granulosa lutein cells and theca lutein cells respectively (luteinization). These cells organize into a highly vascular glandular organ called the corpus luteum (yellow body). The yellow color of this structure is imparted by intracellular accumulations of a pigment, lutein, in some species (equine, bovine, canine and feline). In ewes and sows, other lipid pigments impart the yellow color. If fertilization successfully takes place, the corpus luteum will be maintained. However, if fertilization does not take place, the corpus luteum will degenerate and be replaced by fibrous connective tissue forming the corpus albicans. The corpus luteum is the main source of progesterone, which is important for the maintenance of pregnancy and synergistic with estrogen during estrus.
FIGURE(S): Corpus Luteum – Ovaryhttps://ohiostate.pressbooks.pub/vethisto/chapter/13-the-ovary/
Internal Pelvis, Genitalia, Udder
NOTE: Application terms are bolded, Clinical Notes are bold red, Dissection terms are italicized in this eBook
Application 9 Slides
Application Associated Videos:
Application 9 (1:00:10)
Isolated Boar, Ram, Goat Genitalia (11:45)
Isolated Mare, Cow Genitalia (7:22)
Isolated Male Repro Specimens (4:43)
Mammary Gland (2:20)
Objectives and Terminology Document
Be aware of terminology differences:The uterine tube of anatomy = oviduct of theriogenology and animal science.therio- (Gk.) refers to animals
genology (L.) refers to the science of genesis (reproduction)
The ductus deferens of anatomy = vas deferens of surgery.
A9.1 Describe the normal parts and placement of the lower urinary tract and relationship with the pelvis and perineum.Review of CARNIVORE, as it is similar in large animals:Ureters have a wall with smooth muscle. When the bladder gets full, back pressure on the ureter prevents additional urine from entering the bladder.
The urinary bladder has a wall containing the detrusor m. (smooth m.).
The urethra has an internal urethral sphincter (smooth m.) near the bladder, and an external urethral sphincter/urethralis (skeletal m.) more distal. The pelvic urethra in males and females is covered by the external urethral sphincter/urethralis muscle.
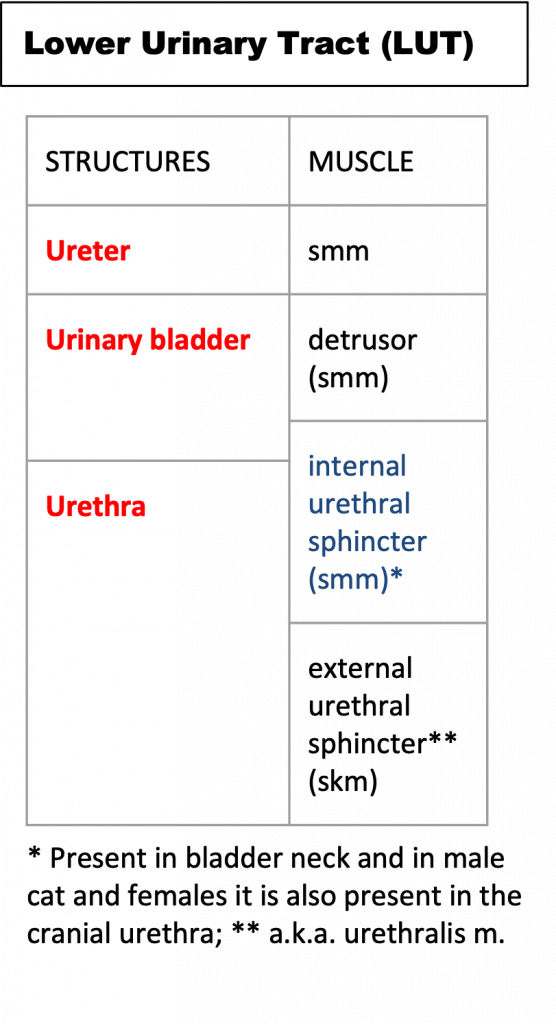
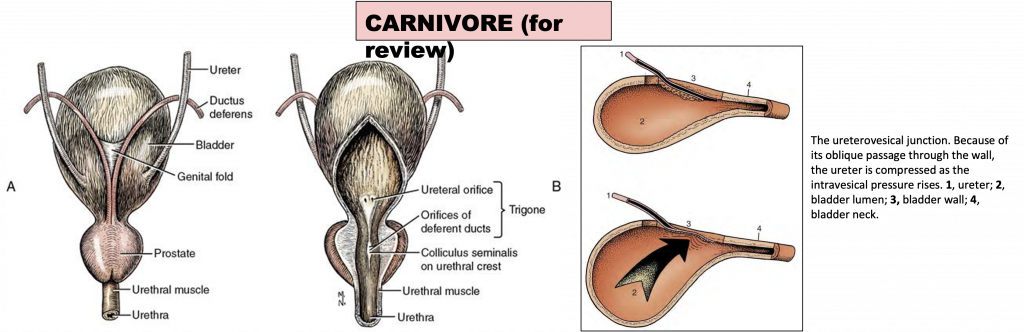
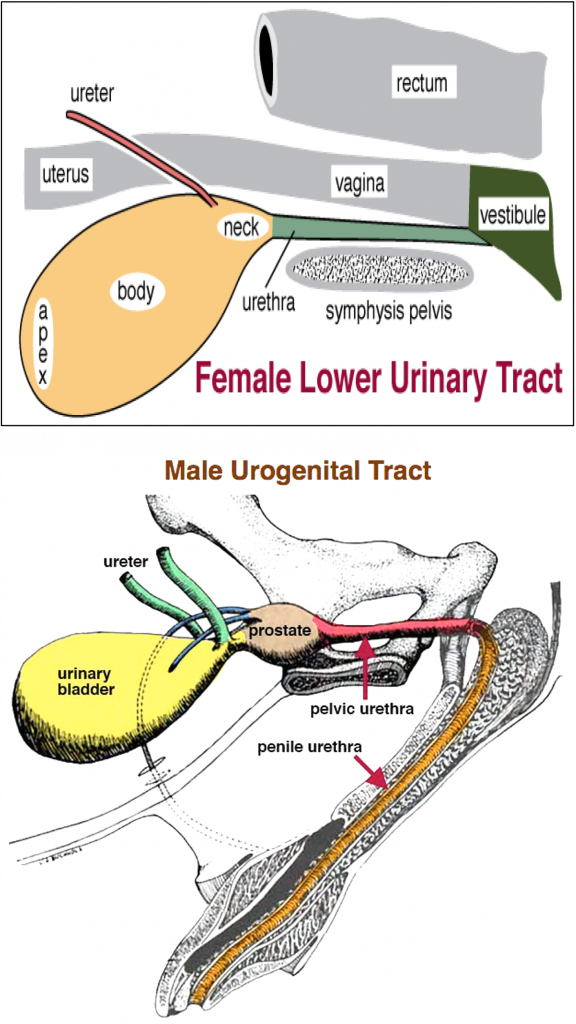
Species differences of the lower urinary tract (LUT) of females (see images below):MARE: urine exits the bladder via the urethra and empties into the vestibule (a common urogenital region in the female). In the pelvis, the bladder is most ventral, next is the reproductive tract, and the rectum is most dorsal.
COW: urine exits the bladder via the urethra, the exit of the urethra into the vestibule is the urethral orifice. Ventral and caudal to the orifice is the suburethral diverticulum.
SOW: urine exits the bladder via the urethra, the exit of the urethra into the vestibule is the urethral orifice. Ventral and caudal to the orifice is the suburethral diverticulum (like in the COW).
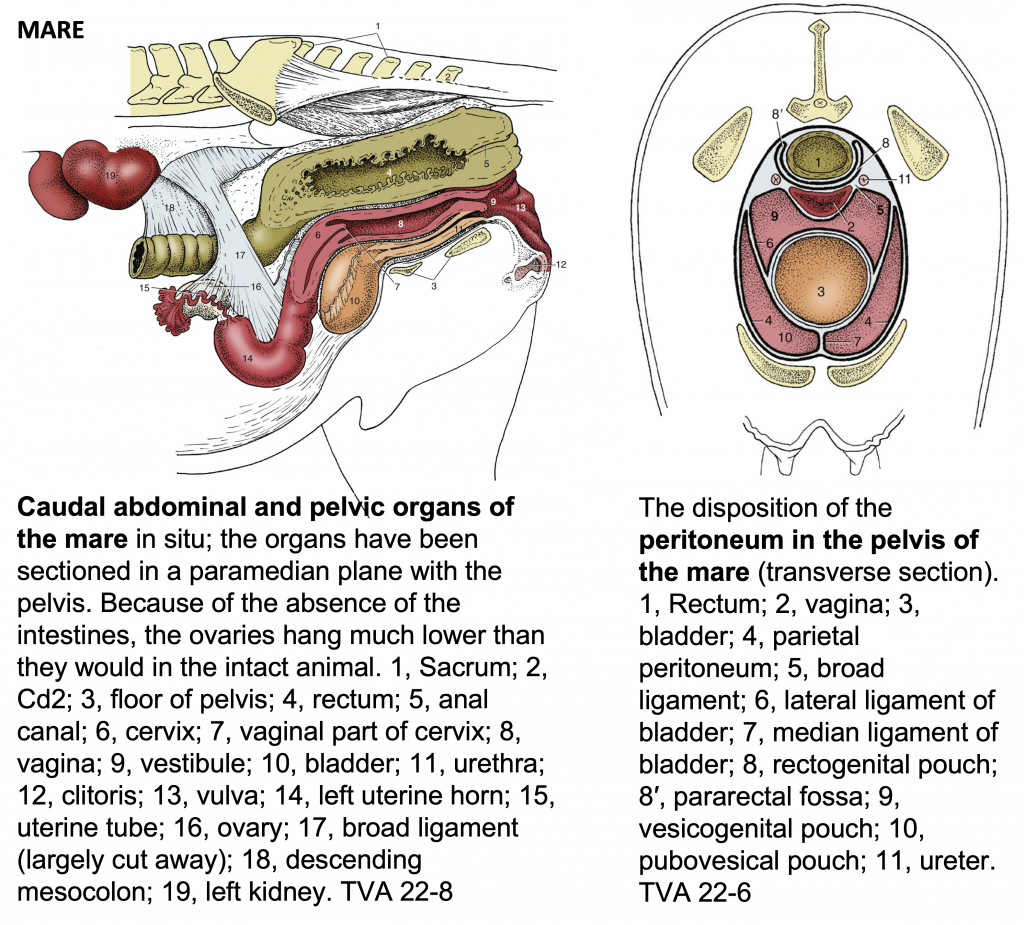
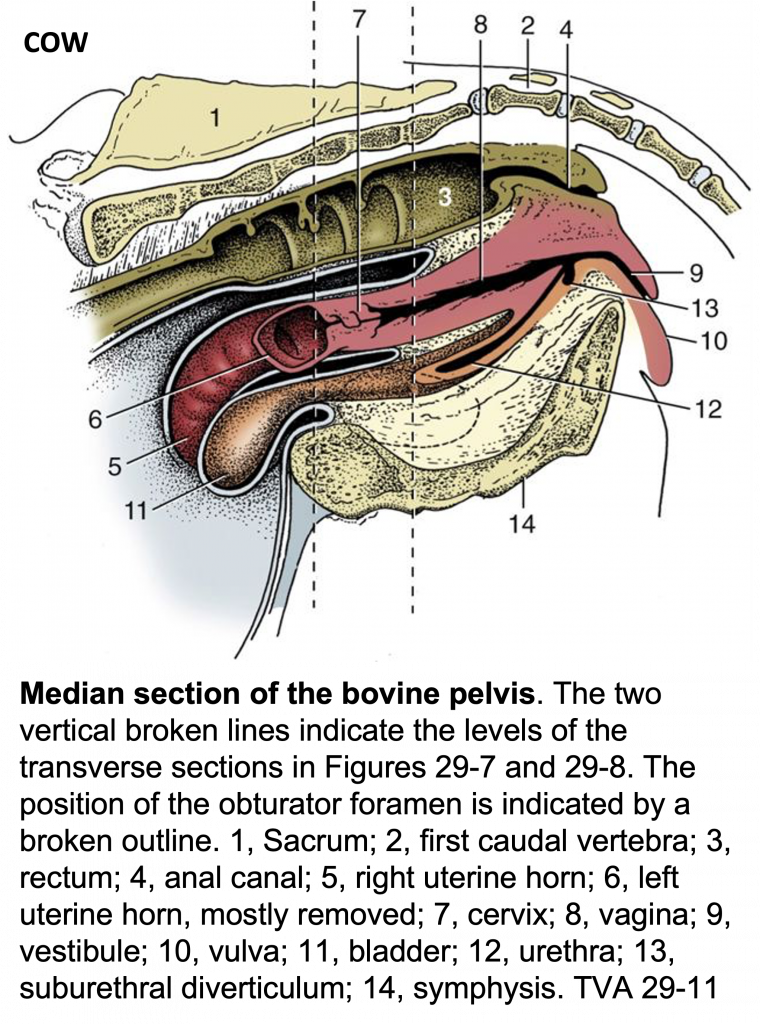
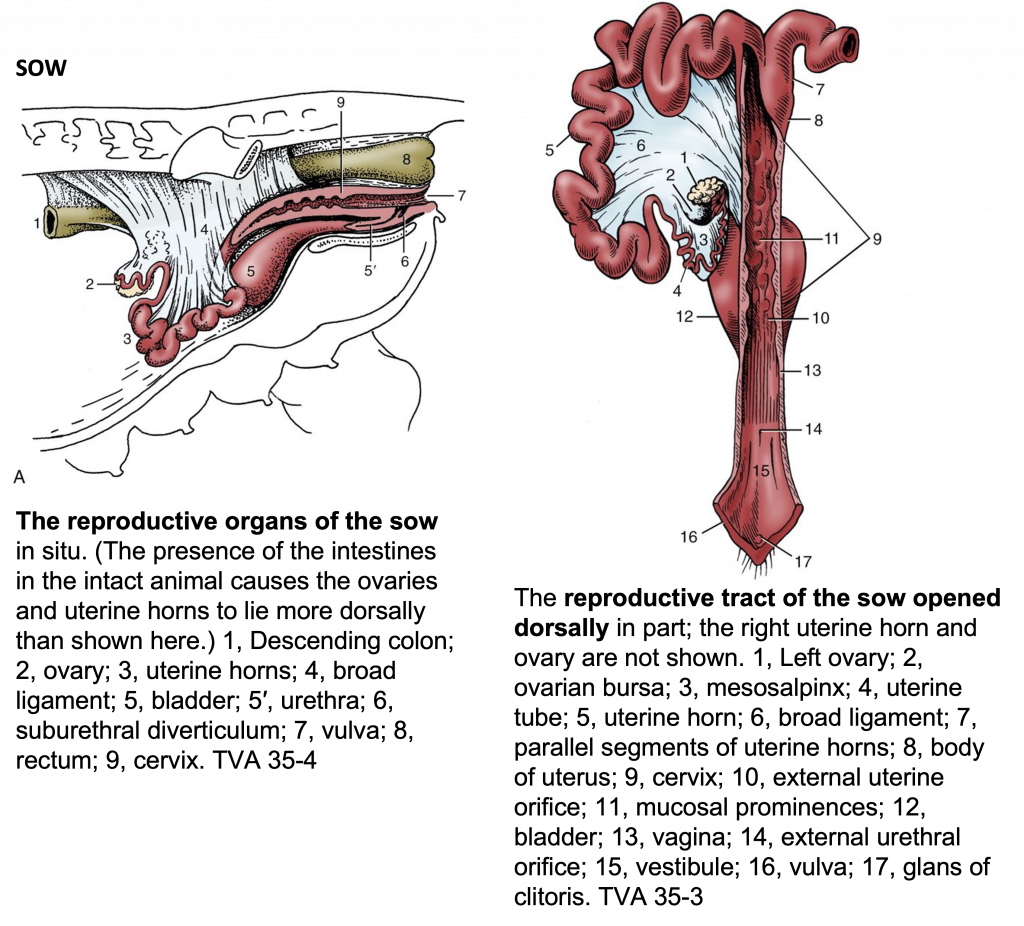
BULL: Urine exits the bladder via the pelvic urethra (surrounded by urethralis m.) first, then passes through the penile urethra to exit the body. There are also several accessory sex glands (e.g., bulbourethral, vesicular, prostate, ampullae) that will add to the seminal fluid. The bulbourethral gland is small, while the prostate is round in BOVINE. In a dorsal view and between the ductus deferens, you can see the genital fold.
BOAR: Urine exits the bladder via the pelvic urethra (surrounded by urethralis m.) first, then passes through the penile urethra to exit the body. There are also several accessory sex glands (e.g., bulbourethral, vesicular, prostate, ampullae) that will add to the seminal fluid. The bulbourethral gland is large, while the prostate is small in PORCINE.
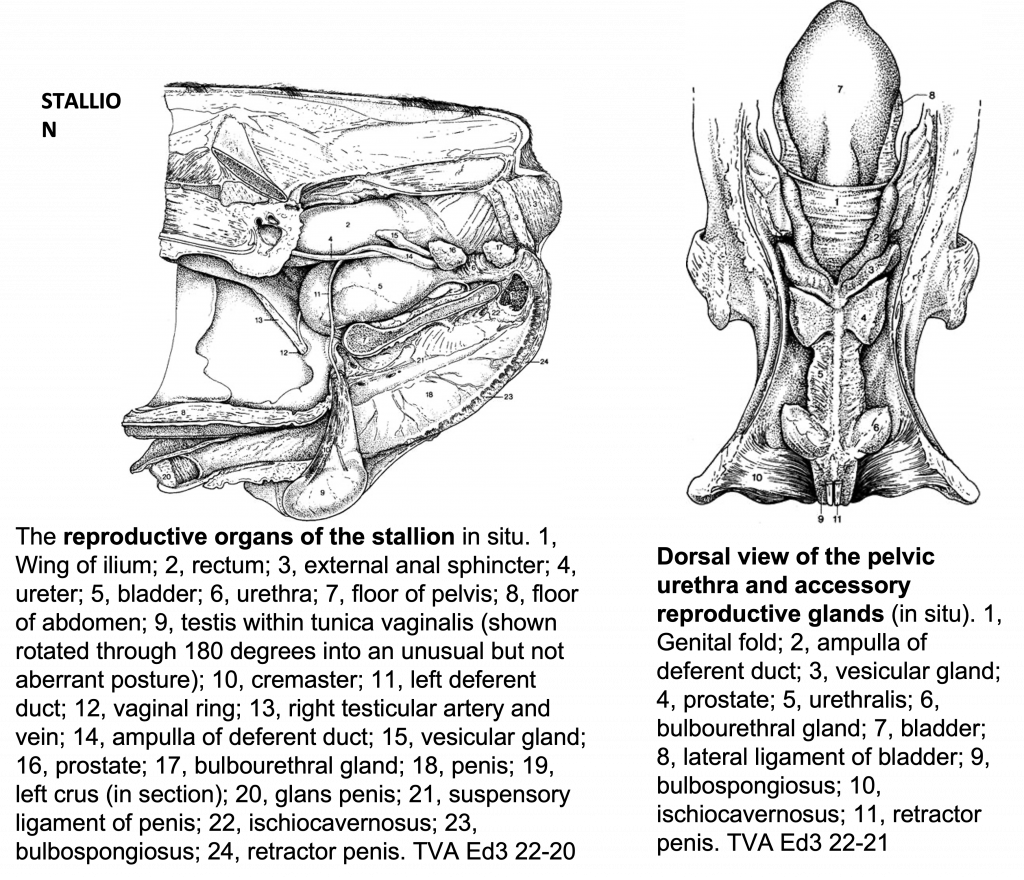
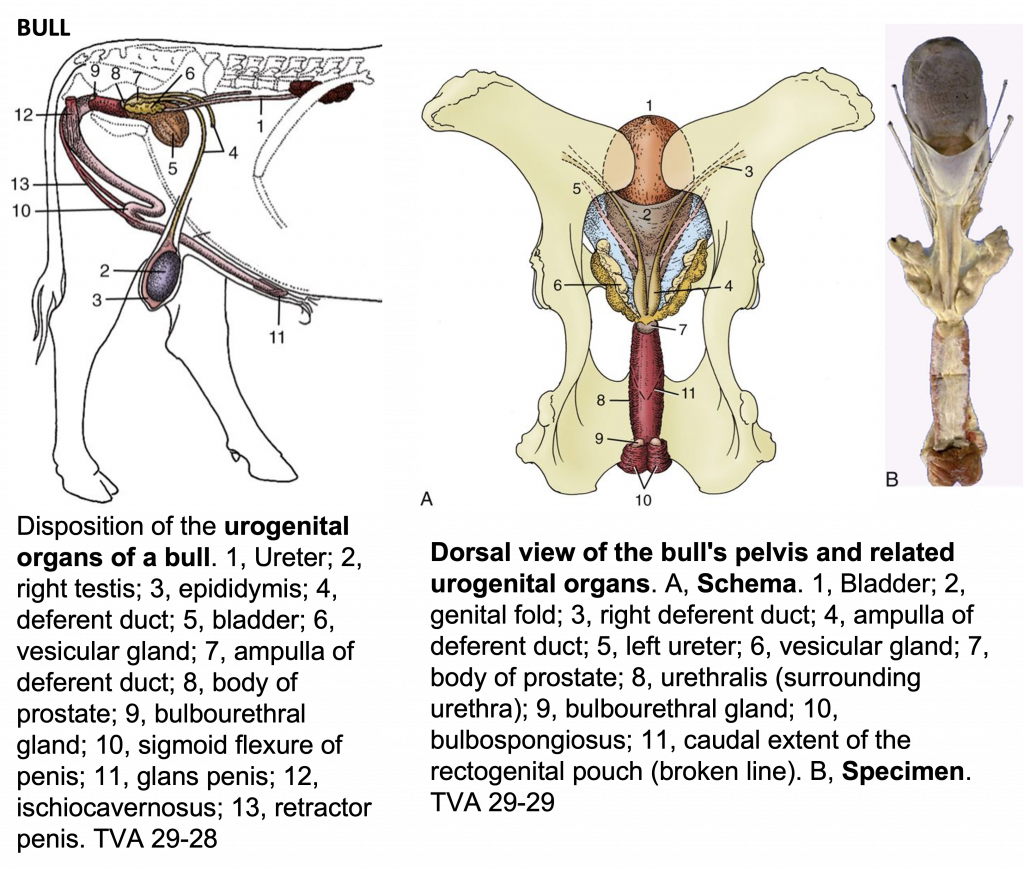
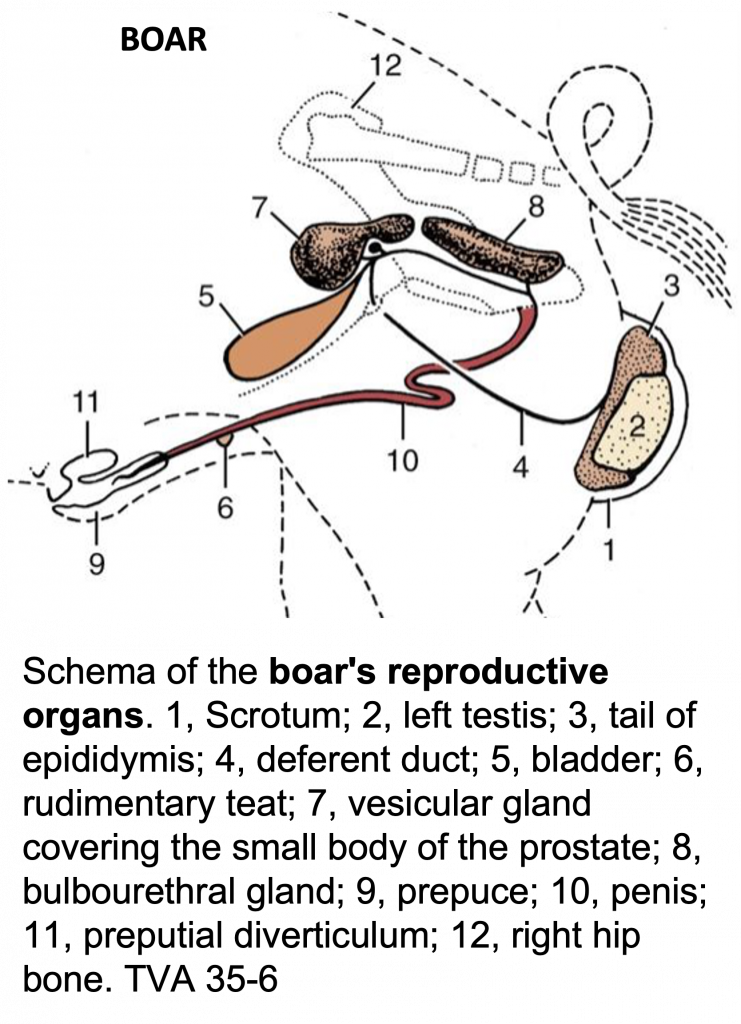
A9.2 Describe and identify the various parts of the male reproductive tract; identify associated structures of the ungulate testis and describe how you would definitively identify a removed/isolated testis as right or left.Make sure you can identify features of the testis (testes/testicles plural) that will help you determine a right from a left testis. This will be important when castrating animals or if they have cryptorchidism.
On a right lateral view (see image below of CANINE testes, A), you will be able to see vessels entering the cranial pole of a testis near the head of the epididymis (the head of the testis is toward the head of the animal). Tubes within the testes produce sperm that enter into the epididymis. The epididymis also has a body, while the tail is near the caudal pole of a testis (toward the tail of the animal).Along the lateral side of the epididymis there is a testicular bursa.
Using the testicular bursa and the position of the head or tail of the epididymis, you should be able to differentiate right and left testicles.
On a medial view (see image below, B), you can see the ductus deferens leading away from the tail of the epididymis. You can also see the vessels entering the cranial pole.
In the cross section of the testis below (C), you can see from outside to inside:skin/scrotum and tunica dartos
external and internal spermatic fascia
parietal vaginal tunic
vaginal cavity
visceral vaginal tunic (on surface of testis)
tunica albuginea
You can also see from the cross sectional view (C) that the vaginal tunic encircles the ductus deferens (called the mesentery of the ductus or mesoductus deferens). The vaginal tunic encircles the testicular vessels (called the mesentery of the vessels or mesorchium).
The cremaster m. (skeletal m.) is one muscle associated with the testis. It is arises from the caudal edge of the internal abdominal oblique m. and courses alongside the spermatic cord. It is in a layer of fascia just outside of the parietal vaginal tunic (cross sectional image below, C). The cremaster m. helps in pulling up the testis (and contracts sporadically).
The tunica dartos (smooth m., found just below the skin of the scrotum) can have a sustained contraction, which helps hold the testis close to the body for warmth. A specific temperature is needed for spermatogenesis. This smooth m. is innervated by postganglionic sympathetic nerves (ilioinguinal n. and posterior scrotal n.).
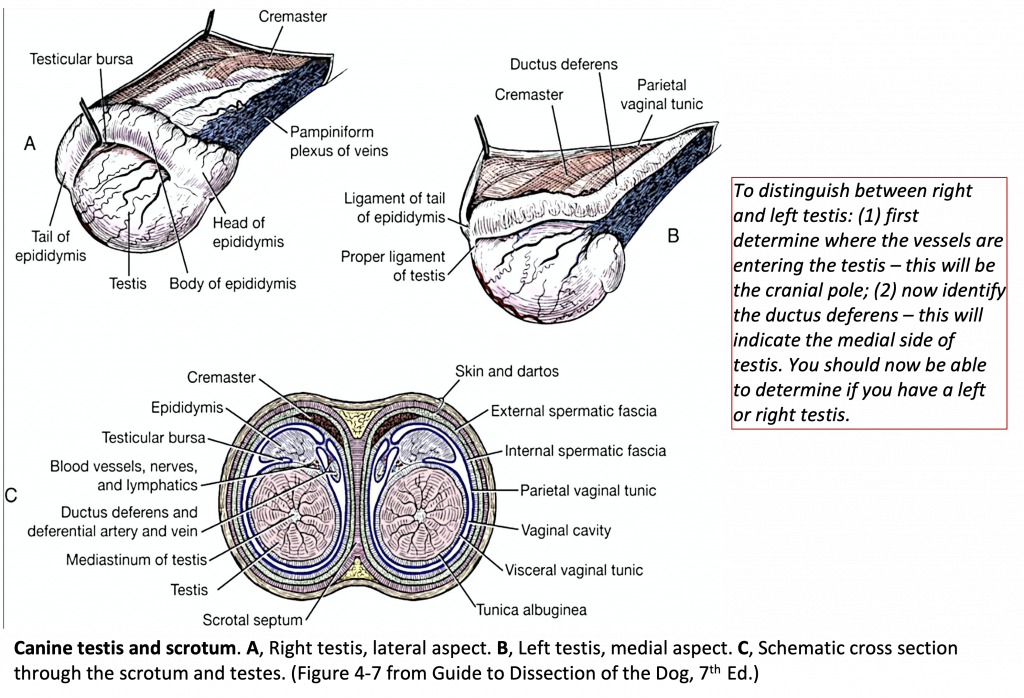
Figure 13-3 Canine testis and scrotum. A, Right testis, lateral aspect. B, Left testis, medial aspect. C, Schematic cross section through the scrotum and testes. (Figure 4-7 from Guide to Dissection of the Dog, 7th Ed.)
The inguinal canal is the opening in the caudal part of the abdominal wall, through which the testis travels in its descent into the scrotum. The canal contains the spermatic cordand external pudendal a. It is a potential space between the flesh of the internal abdominal oblique mm. and the aponeurosis of the external abdominal oblique mm.The entrance (the deep inguinal ring) to the inguinal canal is along the free caudal edge of the internal abdominal oblique m.
The exit (the superficial inguinal ring) is through the aponeurosis of the external abdominal oblique mm..
The peritoneal sheath (i.e., vaginal tunic) of the spermatic cord contains a cavity that places the space about the testis in free communication with the peritoneal cavity of the abdomen. The communication occurs through the vaginal ring (see image below).Clinical Notes: The vaginal cavity provides a possible route for the herniation of intestines that may even reach the scrotum. This occurrence (indirect inguinal hernia) is a comparatively common sequel to castration. Direct inguinal hernia, in which a loop of intestine forces an entry into the canal beside the vaginal tunic, is rare in HORSES.
Incomplete descent of one or both testes (cryptorchidism) is common in the HORSE.
The inguinal canal of CATTLE resembles that of the HORSE. Inguinal hernias are infrequent in CATTLE but common in male SHEEP. (This information about the inguinal region is directly from The Textbook of Veterinary Anatomy, Dyce et al.)
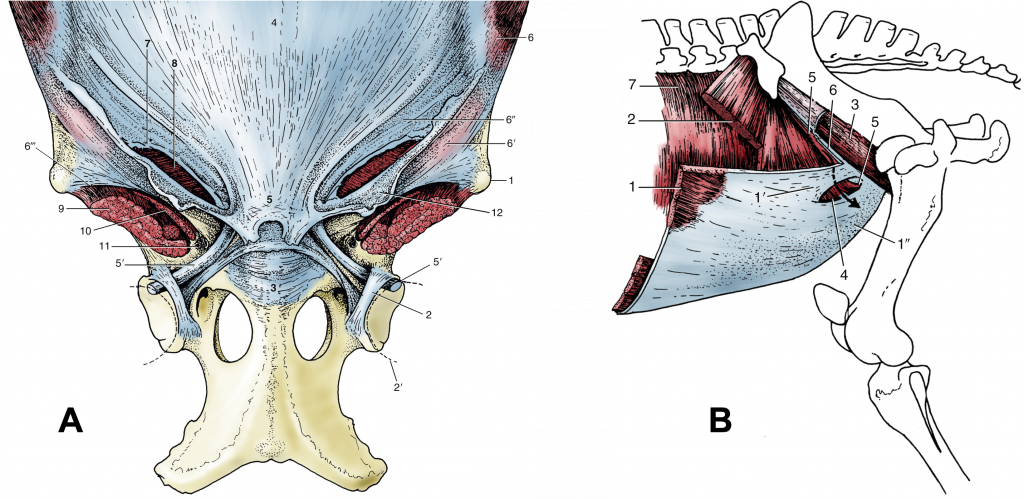
Figure (Above) (A) The attachment of the abdominal muscles on the pelvis and the prepubic tendon. 1, Coxal tuber; 2, transverse acetabular ligament; 2′, femoral head; 3, pubis; 4, tunica flava over linea alba; 5,prepubic tendon; 5′, accessory ligament; 6, external abdominal oblique; 6′ and 6″, pelvic and abdominal tendons of external oblique aponeurosis, respectively; 6′″, attachment of pelvic tendon of external oblique aponeurosis on sartorius and iliopsoas (“inguinal ligament”); 7, superficial inguinal ring; 8, internal abdominal oblique; 9, iliopsoas; 10, sartorius; 11, vascular lacuna containing femoral vessels; 12, femoral fascia (lamina).
(B) The muscles of the inguinal region. The arrow passes through the inguinal canal. 1, External abdominal oblique; 1′ and 1″, pelvic and abdominal tendons of external oblique aponeurosis, respectively; 2, internal abdominal oblique; 3, iliopsoas partly enclosed by iliac fascia; 4, superficial inguinal ring; 5, cranial border of deep inguinal ring; 6, attachment of pelvic tendon of external oblique aponeurosis on iliopsoas and sartorius (“inguinal ligament”); 7, transversus abdominis.
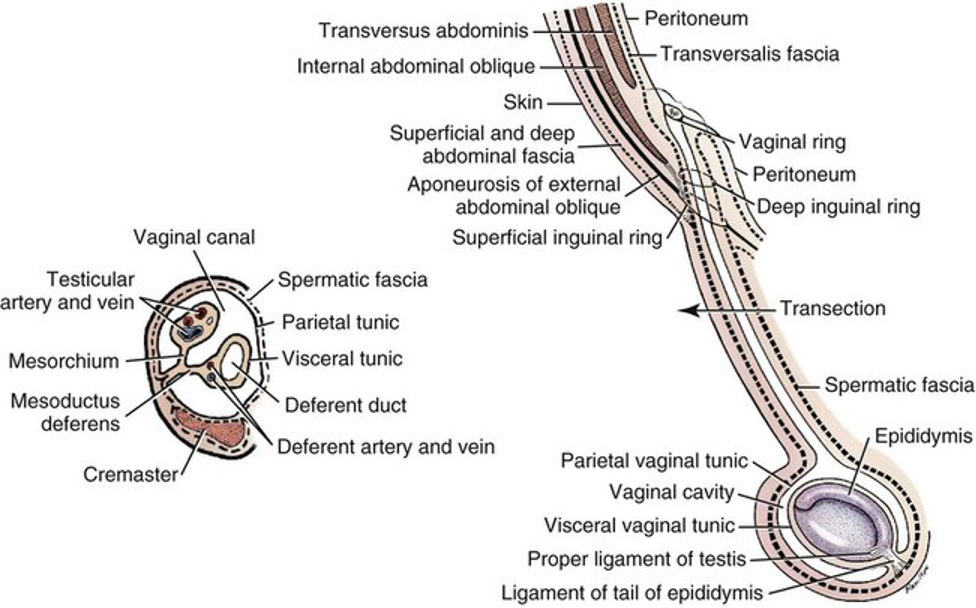
A9.3 Identify and describe the gross morphology and basic functional role of the accessory sex glands in the stallion, bull, and boar; summarize differences seen amongst these species and compare/contrast with the carnivore.Note the presence or absence of particular accessory sex glands in various (male) species: stallion/eq, bull/bov, boar/por, canine. Also be able to describe the general function or role the the glands. All glands are paired (i.e., bilateral) except the prostate.
The types of glands include (each species is described further below): Prostate (present in all domestic mammal species)
Ampullae/ampullary (present in ruminants, horses, dogs; absent in cats; poorly developed in the boar); it is typically a widening or enlargement of the distal ducts deferens.
Vesicular/seminal vesicles (present in some form in horses, ruminants, swine; absent in the dog and cat)
Bulbourethral (present in all domestic mammal species except the dog).
The table below helps to clarify the presence of each gland and if there are size or shape differences in the various species.
The function or role of the accessory sex glands is to contribute to ejaculate or seminal fluid (the fluid component of semen for sperm to travel with during ejaculation). Each contributes to varying degrees, and can be particular to a species.
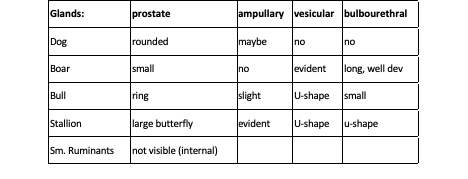
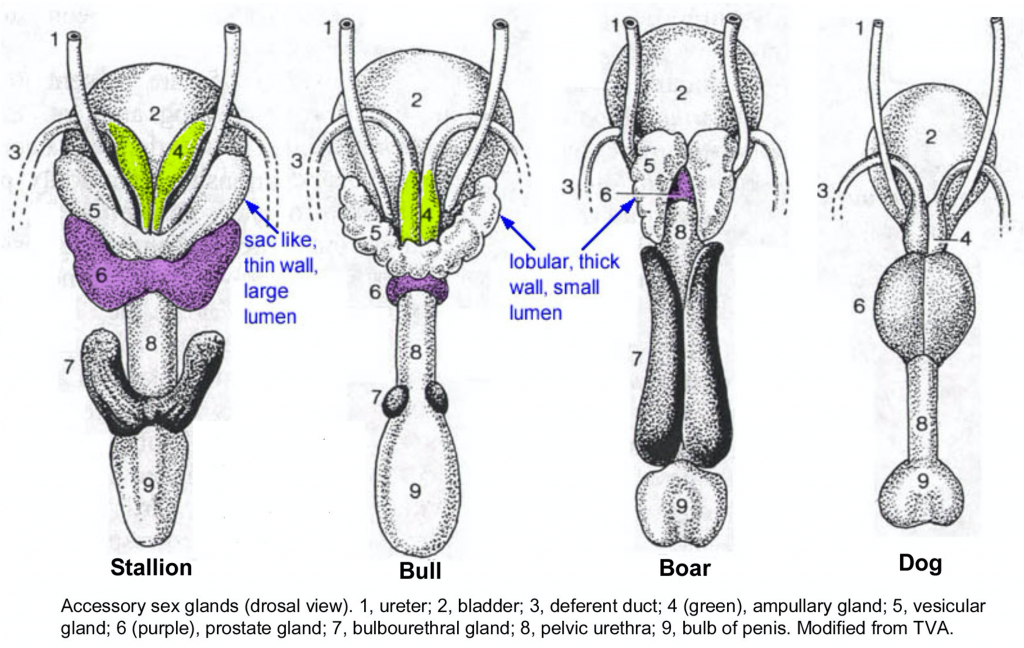
EQUINE Stallion: Accessory Sex Glands (Figure 13-4 and image above)Genital fold: this is the membrane connecting the ductus deferens. Within this fold there may be a remnant of the paramesonephric ducts, the uterus masculinus. If present, it will appear as a midline thickening.
Ductus deferens (one from each testis): each ductus deferens will have a distal enlargement, the ampulla (ampullary gland).
Vesicular glands/seminal vesicles (paired): the vesicular glands are smooth, sack-like structures lateral to the ampullae.
Body of the prostate: the prostate is unpaired and surrounds the urethra in the region caudal to the ampullae and vesicular glands.
Urethralis muscle: this is a tough sphincter surrounding the urethra that is responsible for urinary continence (prevention of urine flow).
Bulbourethral glands (paired): the bulbourethral glands are partially covered with skeletal mm. (it may be derived from the urethralis or bulbospongiosus mm. depending on species) and are found at the ischial arch.
You can also see in the images below, the bulbospongiosus m. (along the bulb of the penis) and ischiocavernosus m. (at the root of the penis, lateral to bulb, and over the crura).
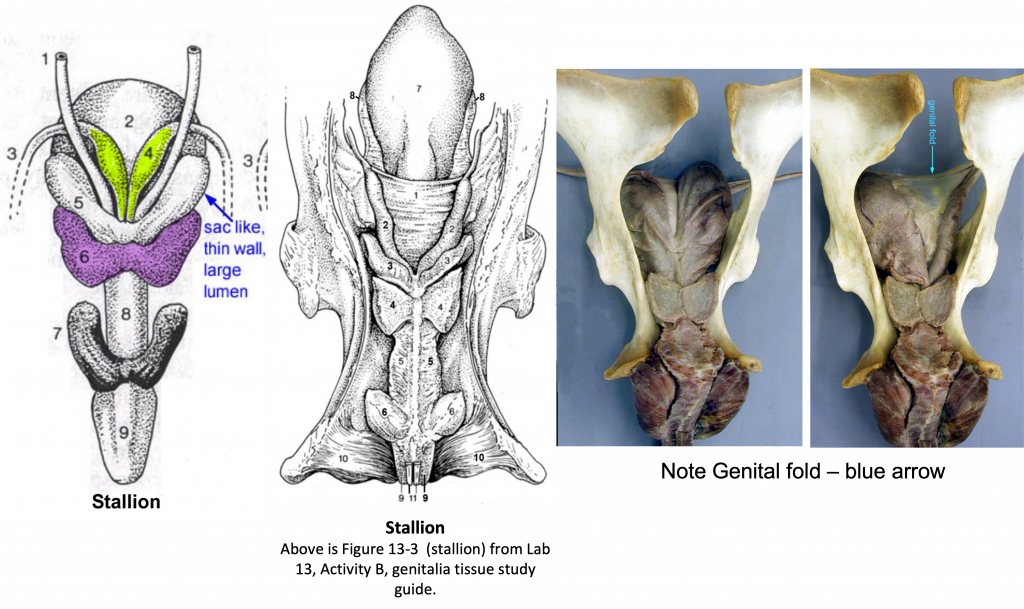
Figure 13-4 (Above, center) Equine male genitalia. 1, genital fold; 2, ampullae of the ductus deferens; 3, vesicular glands; 4, body of the prostate gland; 5, urethralis muscle; 6, bulbourethral glands; 7, bladder; 8, lateral ligament of the bladder; 9, bulbospongiosus m.; 10, ischiocavernosus m. (covering the crus of the penis); 11, retractor penis m.; 12, urethral mucosa; 13, corpus spongiosum; 14, tunica albuginea; 15, corpus cavernosum.
RUMINANT (bovine, caprine, ovine): Accessory GlandsAmpulla(e)/ampullary glands (paired): similar to the HORSE, the ampulla is a distal enlargement of the ductus deferens with a glandular wall.
Vesicular glands (paired): vesicular glands are lobular and adjacent to the insertion of the ampullae. They produce the majority of the seminal fluid in the BULL. These glands are more than twice as large in the BULL as they are in a STEER (castrated male ox/cattle).
Prostate gland (unpaired): the prostate is small in the BOVINE and not grossly visible in small RUMINANTS (i.e., it consists of only internal glandular tissue).
Bulbourethral glands (paired): these glands are small in RUMINANTS.
PORCINE: Porcine Accessory Glands
Special Notes: While boar testes are similar to other species the tail of the epididymis is very large in BOARS. The accessory glands are large in the BOAR but small in the BARROW (castrated male pig).Vesicular glands (paired): the vesicular glands are very large in the BOAR.
Prostate (unpaired): the prostate may be hidden from view by the large vesicular glands.
Bulbourethral glands (paired): the bulbourethral glands are large, and furnish the watery pre-sperm fraction that washes the urine out of the urethra before the sperm-rich fraction is passed.
A9.4 Describe the general topography of extrinsic muscles of the penis, erectile tissues (with associated connective tissue), and penile urethra in ungulate species; identify these structures on images and specimens.In the ventral views of pelvis and penis below, you can see red indicates the corpus cavernosum erectile tissue. Once all of the tissues of the penis are together, there is a root, body, and free part of the penis. The free part is the portion within the prepuce.
The root is formed by the two crura (which are attached to the ischial arch). Once the crura combine they form a common body.
The crura are covered by the ishiocavernosus m.
The corpus cavernosum erectile tissue is surrounded by a dense tunica albuginea.
The orange indicates the corpus spongiosum erectile tissue.The bulb is near the ischial arch and is covered by the bulbospongiosus m.
Distally, the corpus spongiosum expands to form the glans penis.
The corpus spongiosum surrounds the penile urethra along the length of the penis. The corpus spongiosum glandis is located at the distal portion (the glans penis).
The extrinsic muscles of the penis (ishiocavernosus, bulbospongiosus mm.; skeletal mm.) aid in erection, generating higher pressure within penis for ejaculation.
Also note the bilateral smooth muscle, the retractor penis m.
More details on each species are in the next section.
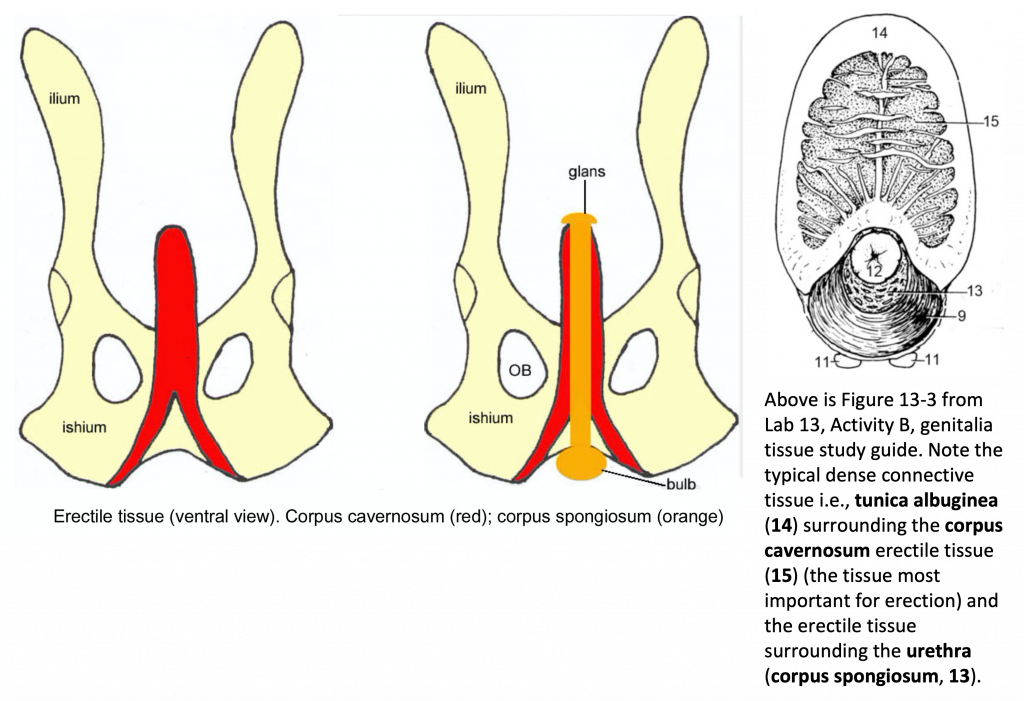
Clinical Note: the male urethra in RUMINANTS and SWINE has a urethral diverticulum/recess (see image below, from Farm Animal Surgery) that extends from the dorsum of the urethra (intersection of the pelvic and penile urethra) at the level of the ischium. This recess can make the passage of a urinary catheter into the bladderdifficult. During retrograde urethral catheterization, it almost invariably enters the recess and cannot be redirected into the bladder. To confirm that the catheter has reached the urethral recess, the surgeon can introduce a hand or finger into the animal’s rectum and palpate the catheter tip at the caudal aspect of the pelvic urethra while the catheter is gently moved back and forth.
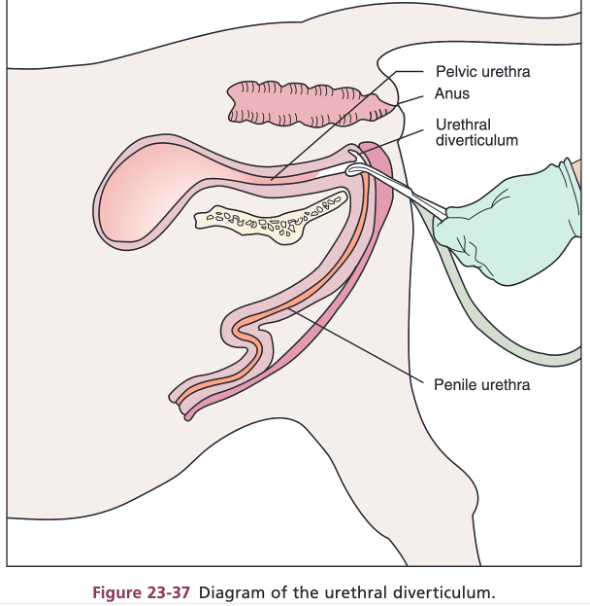
A9.5 Compare form and function of the equine, bovine, and porcine penis; describe and identify distinctive features of the penis and prepuce in these animals.Vascular penis: erectile tissue expands the length and diameter of the penis (e.g., equine) (see image below).STALLION – the distal end (glans penis) of the penis greatly expands, and contains corpus spongiosum glandis (erectile tissue). Equine have a urethral process(protrusion on the tip of the penis), which is surrounded by a moat called the fossa glandis. The urethral sinus is a dorsal diverticulum of the fossa glandis, where debris can collect (called ‘bean’). This debris is hardened accumulation of smegma (secretion produced by glands in the preputial lining), and sedation is used to remove it.
Fibrous penis: the erectile tissue is within a fibrous tube and can’t expand in diameter. The erectile tissue mostly straightens out the penis (specifically at the sigmoid flexure) (e.g., bovine, porcine, small ruminant) (see image below).RAM, GOAT – these small ruminants have a glans penis with corpus spongiosum glandis as well as a very long urethral process. Unfortunately, the long and narrow urethral process can be a point where urinary calculi can get stuck.
BULL – the urethral process in the bull is not as long as the other ruminants.
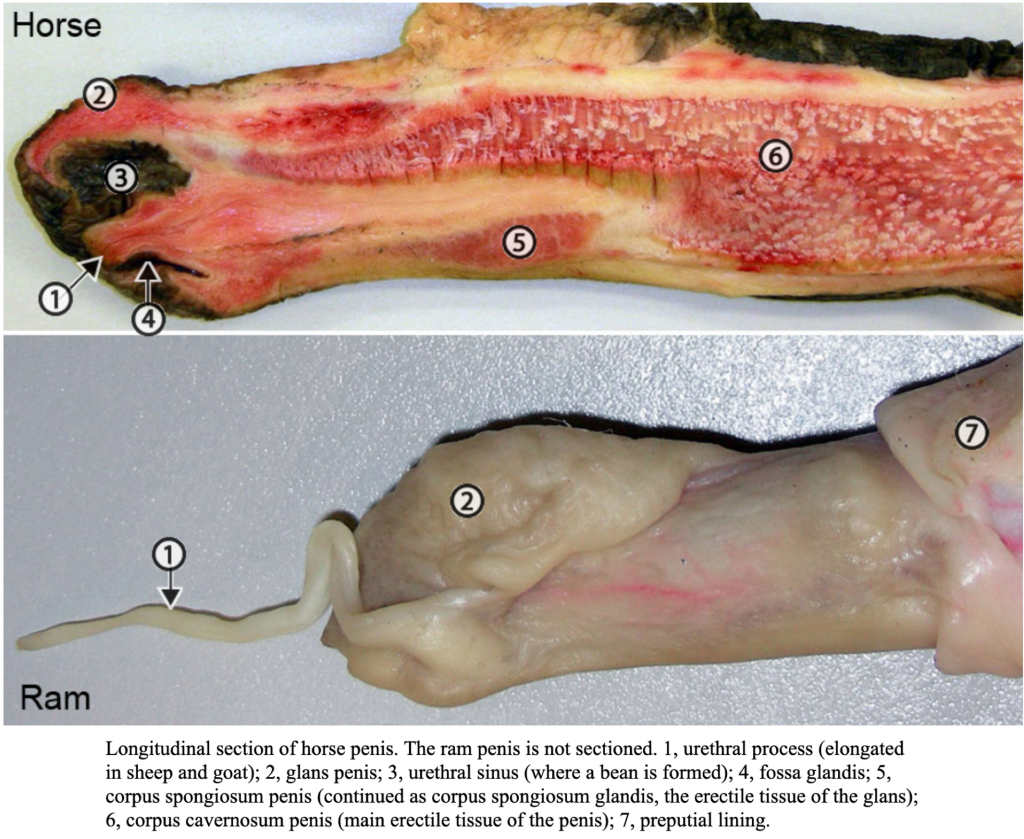
The prepuce is an external layer of skin that covers the penis.In EQUINE, the prepuce has an internal and external layer. The external layer covers the penis, while the internal layer covers the next segment of the penis as it becomes more erect. (Figure 13-5)Preputial orifice: this is the external opening of the prepuce where hairy external skin meets the preputial lining.
Preputial ring: this is the free folded edge of the inner layer of the prepuce. When the penis is erect, the preputial ring appears as a raised ridge going around the penis.
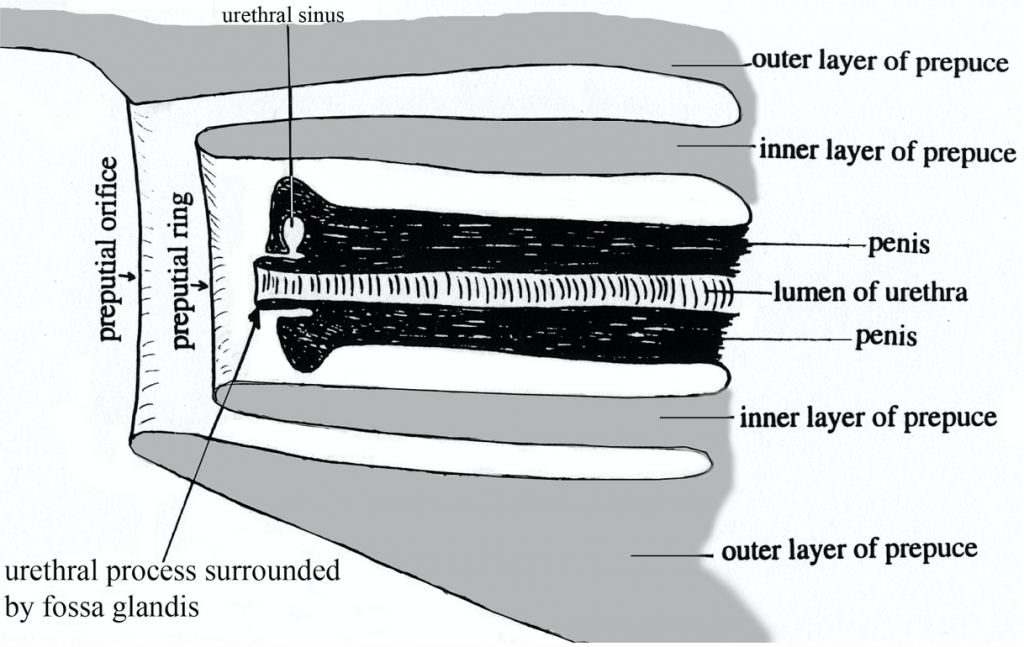
Figure 13-5 (Above) Schematic median section of equine penis and prepuce.
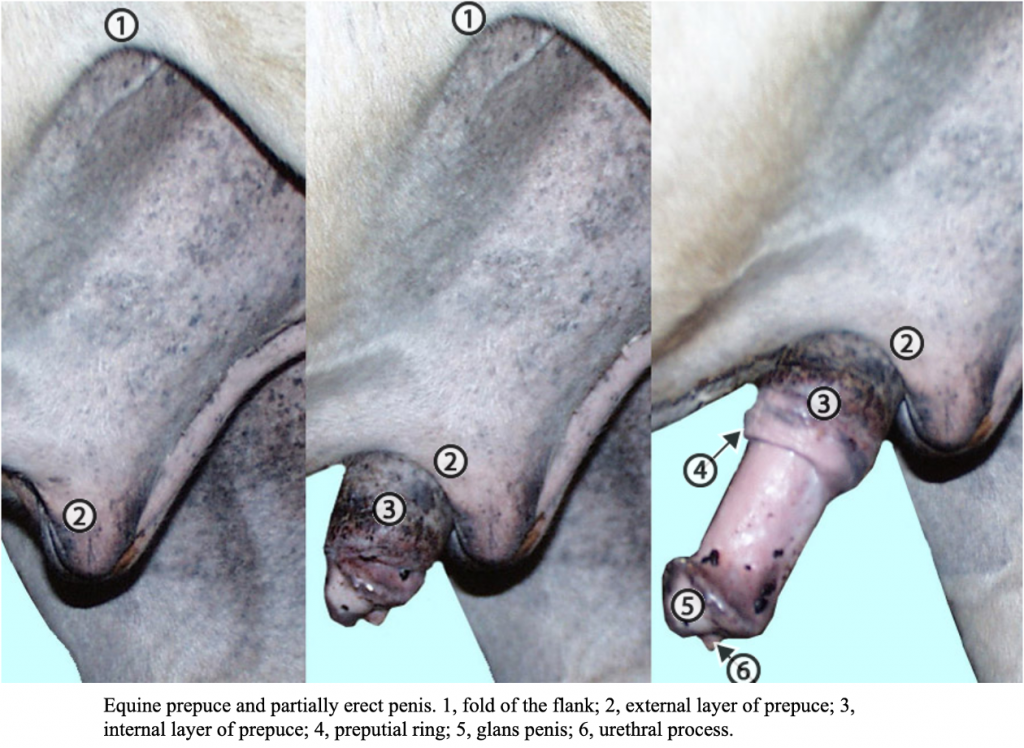
In BOVINE and PORCINE, the prepuce isn’t as extensive (as in the HORSE). In the image below, you can see a dissected view of the prepuce showing the glans and free part of the BOVINE penis.
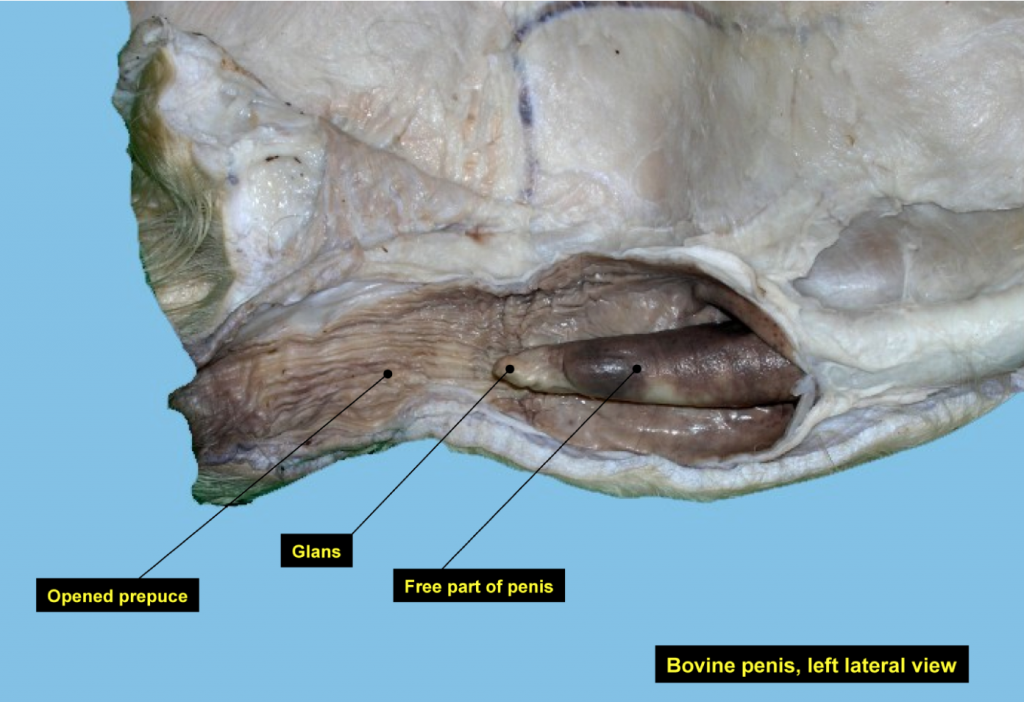
Equine Penis (Figure 13-4)Root of the penis: the root is the proximal part of the penis with laterally positioned crura (left and right).Each crus is composed of erectile tissue (corpus cavernosum penis) encased with a fibrous tunic (tunica albuginea), which serves to anchor each side of the penis to the ischial arch; the crura are covered by ischiocavernosus m.
Medial to the crura, the expanded erectile tissue (corpus spongiosum penis), aka bulb of the penis, is also surrounded by muscle, the bulbospongiosus m. In the HORSE, this muscle extends most of the length of the penis.
The more superficial, paired, retractor penis mm. extend the length of the penis.
Body of the penis: the body begins at the region where the bilateral crura meet and then extends distally to the free part.
Free part of the penis: this is the portion of the penis within the prepuce, including the distal glans penis (with corpus spongiosum glandis) (the portion of the penis that is shaped like a ‘bell’ when engorged).
The dorsal a. of the penis is the main blood supply for the penis. The internal pudendal a. gives rise to the dorsal a. of the penis, while the obturator a. also serves as a blood supply to the penis in the HORSE.
The dorsal n. of the penis is found along the dorsal midline of the penis. The pudendal n. provides this branch.
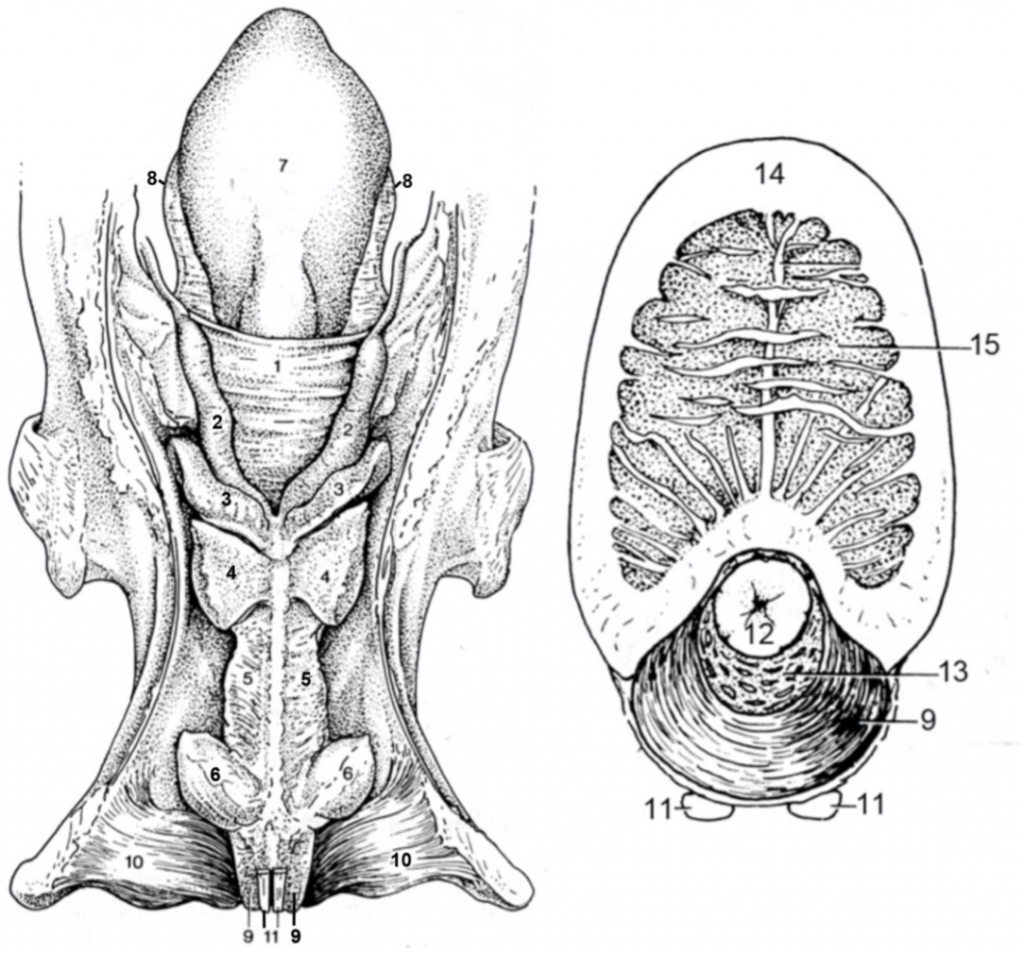
Figure 13-4 (Above) Equine male genitalia. Left: dorsal view of genital tract; Right: cross section of penis. 1, genital fold; 2, ampullae of the ductus deferens; 3, vesicular glands;4, body of the prostate gland; 5, urethralis muscle; 6, bulbourethral glands; 7, bladder; 8, lateral ligament of the bladder; 9, bulbospongiosus m.; 10, ischiocavernosus m. (covering the crus of the penis); 11, retractor penis m.; 12, urethral mucosa; 13, corpus spongiosum; 14, tunica albuginea; 15, corpus cavernosum.
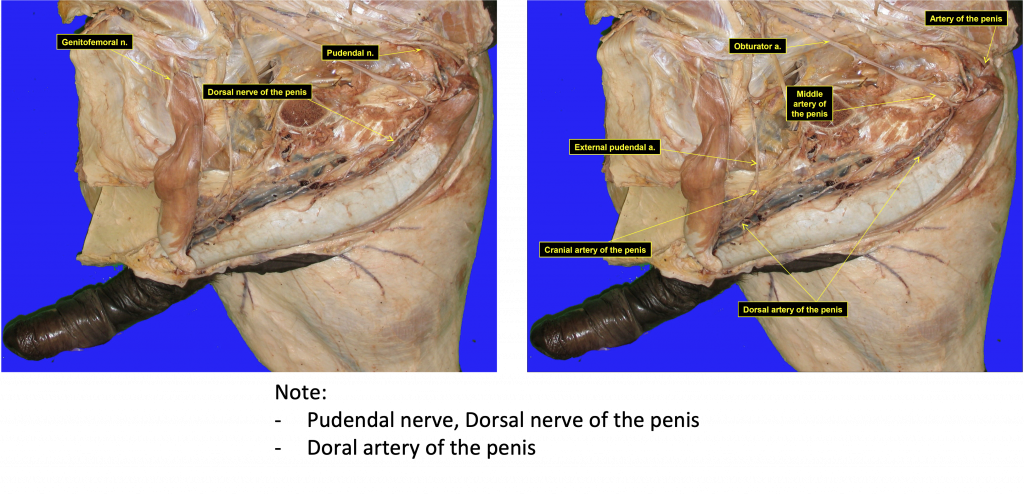
Ruminant Penis (Figure 13-6)Root of the penis: the root is the proximal part of the penis with laterally positioned crura (left and right).Bulbospongiosus m.: the striated muscle covering the midline portion of the root of penis. Similar to other species, the urethra is surrounded by the corpus spongiosum penis erectile tissue which in turn is covered by the bulbospongiosus m.
Ischiocavernosus m.: the striated muscle covering the two crura that anchor the root of the penis to the ischial arch. Each crus of the penis is composed of corpus cavernosum penis erectile tissue (within a sleeve of tunica albuginea) which in turn is covered by the ischiocavernosus m.In a cross sectional view of a crus, the surrounding white tunica albuginea and darker erectile tissue is sometimes called ‘pizzle eye.’There are also the superficial, paired, retractor penis mm. extending down the midline of the penis.Body of the penis: the body begins at the region where the bilateral crura meet and then extends distally to the free part.In RUMINANTS, the body of the penis is curved into the sigmoid flexure which is composed of a proximal loop and a distal loop; note the smooth curvature of the proximal loop and the acute bend that forms the distal loop. The acute angle increases the likelihood that urinary calculi will get stuck.
Note that the retractor penis m. inserts beyond the distal loop, but is free from the proximal loop.
Free part of the penis: the portion of the penis within the prepuce including the distal glans penis (with corpus spongiosum glandis).Note the relatively small glans penis and the tubular urethral process, which is fused to the glans. The urethral process of the RAM and BILLY are elongated and free.
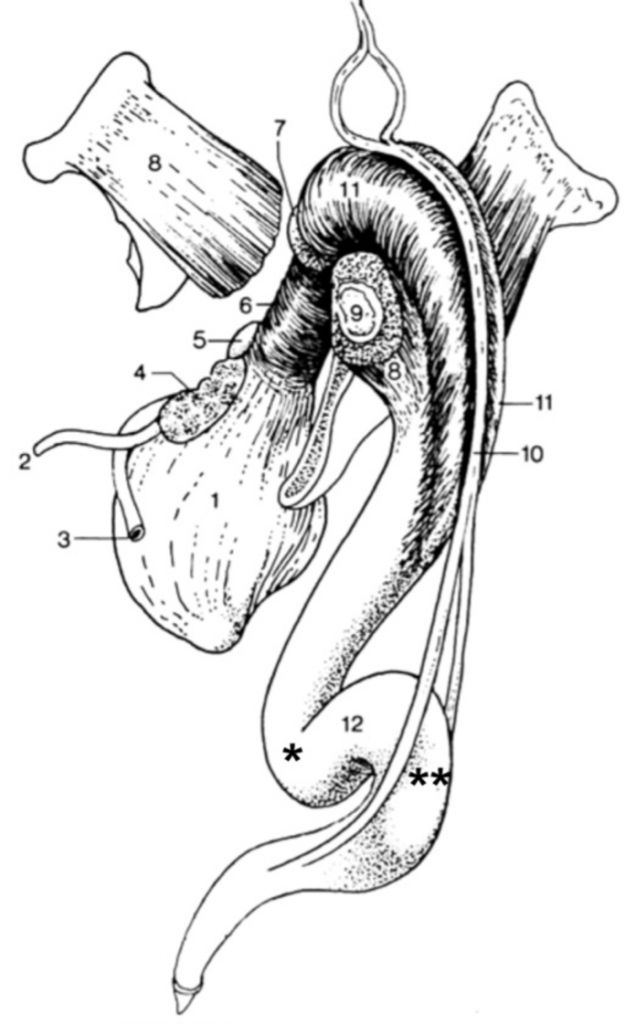
Figure 13-6 (Above) Bovine male genitalia, caudal lateral view; part of the left pelvis has been removed. 1, urinary bladder; 2, ureter; 3, ductus deferens; 4, vesicular gland; 5, prostate gland; 6, urethralis m.; 7, bulbourethral gland; 8, ischiocavernosus m., covering the crus of the penis (9); 10, retractor penis muscle; 11, bulbospongiosus m.; 12, sigmoid flexure of the penis, formed by proximal (*) and distal loops (**). (Duplication of TVA Fig. 29-33).
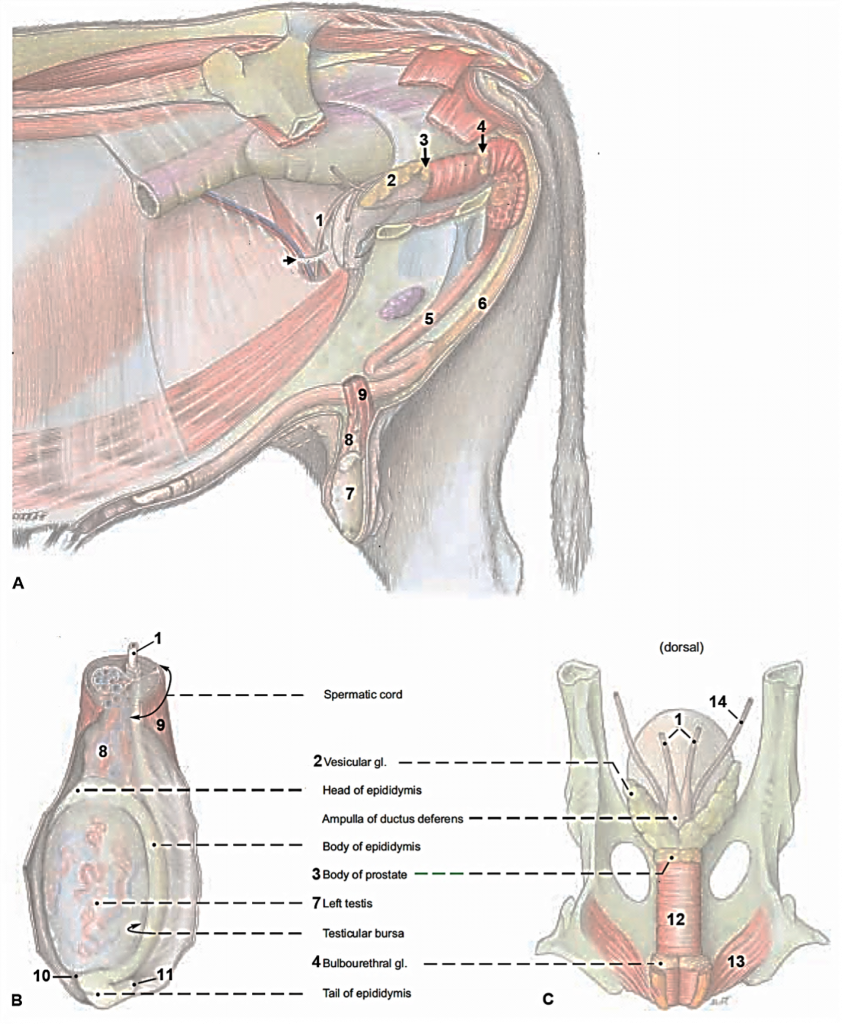
Figure 13B-1 A. Bull reproductive tract, left limb and body wall removed; B. left testis(lateral view); C. urogenital tract (pelvic portion; dorsal view). 1. ductus deferens, 2 vesicular gland; 3, prostate, 4, bulbourethral gland; 5, penis; 6, retractor penis m.; 7, left testis; 8, testicular a. and vv.; 9, cremaster m.; 10, proper ligament of testis; 11, ligament of the tail of the epididymis; 12, urethralis m.; 13, ischiocavernosus m.; 14, ureter; arrow, vaginal ring (right side). (Budras)
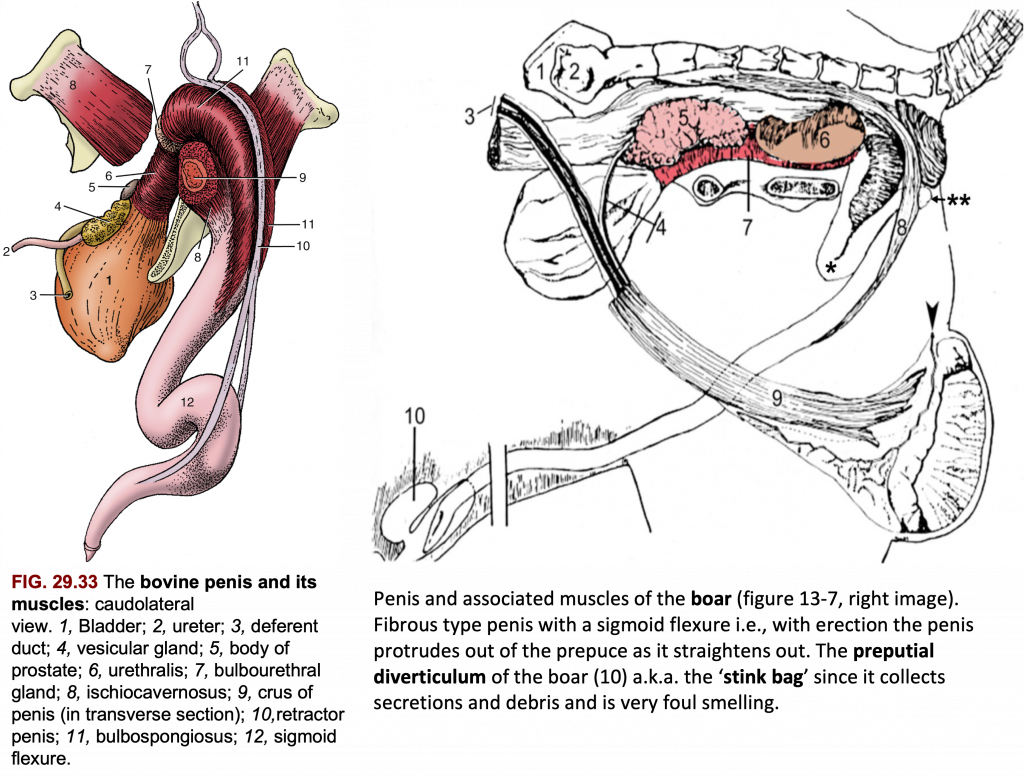
Figures – Bovine (left), Porcine (right)
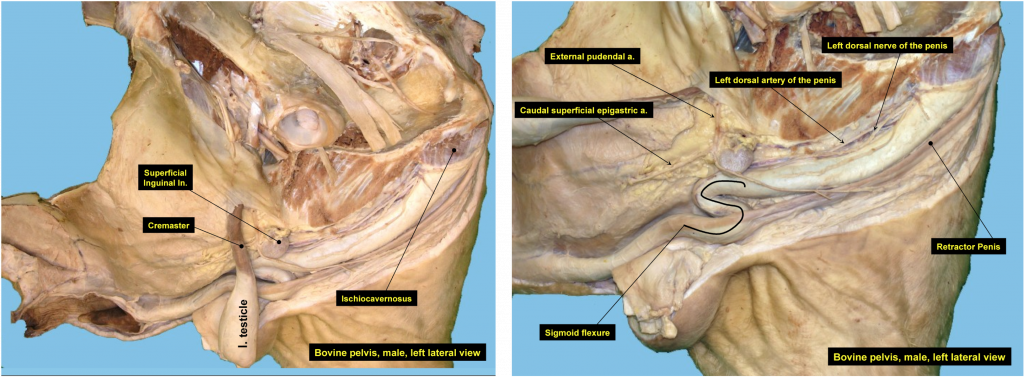
Figures – Bull, midline views
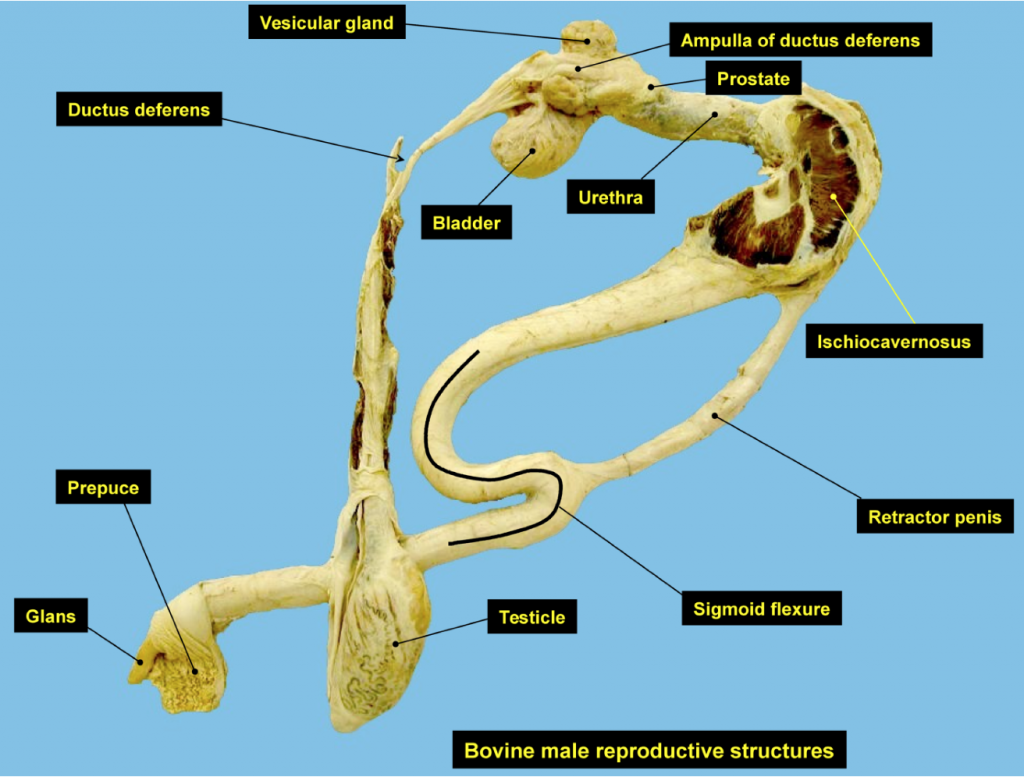
Figure – Isolated bull reproductive tract
Porcine Penis (Figure 13B-2)Sigmoid flexure: the sigmoid flexure of the boar penis usually lies at the level of the testes. Similar to the RUMINANT, the sigmoid flexure of the BOAR is composed of a proximal loop and a distal loop.
Note that the end of the BOAR penis has a curvature which becomes corkscrew shaped when erect.
Near the preputial orifice find the opening of the preputial diverticulum (aka ‘stink bag‘). As it’s name implies, it collects smelly debris.
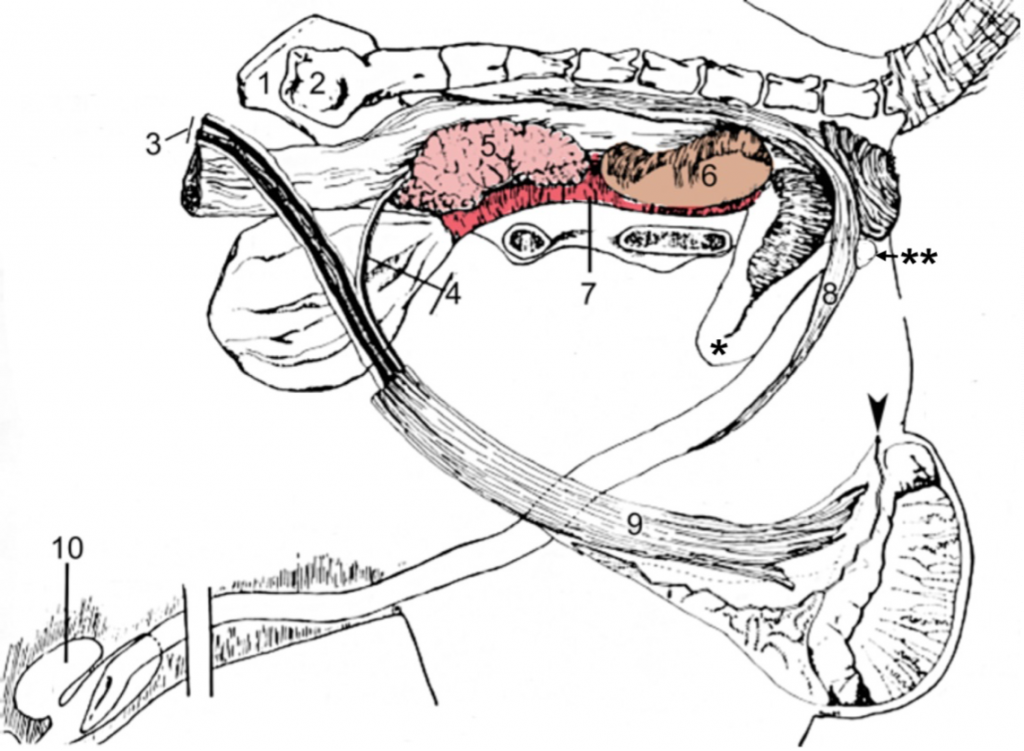
Figure 13B-2 Genitalia of a boar (left limb and hemipelvis removed). 1, right wing of ilium; 2, left wing of sacrum; 3, testicular vessels; 4, ductus deferens and associated vessels; 5, vesicular gland (obscures view of prostate gland); 6, bulbourethral gland; 7, urethralis m. (skeletal muscle); 8, retractor penis m. (smooth muscle); 9, cremaster m. (skeletal muscle) of left testis; 10, preputial diverticulum (‘stink bag’), arrowhead, cut edge of vaginal tunic; proximal loop (*) and distal loop (**) of sigmoid flexure of penis.
A9.6 Describe and identify the various parts of the female reproductive tract; compare/contrast the reproductive tracts of the mare, cow, and sow and identify features of clinical significance.
FEMALE GENITALIA: All species
Ovary and Uterine Tube: Ovary: the ovaries are rounded and firm. There are no nerves that enter the ovary except those associated with blood vessels.
Ovarian bursa: the bursa is formed by the mesovarium and the mesosalpinx lateral and cranial to the ovary. This bursa communicates with the peritoneal cavity.The mesovarium and mesosalpinx, along with the mesometrium, make up the broad ligament of the uterus.Uterine tube (aka oviduct): the tube runs in the mesosalpinx from its opening, the infundibulum, around the cranial aspect of the ovary, to the lateral surface, and then to the uterine horn.The uterine tube is thrown into a tight wave-like path by its muscular walls.
Note how the uterine tube begins as a wide structure (i.e., ampulla) and tapers to a uniform diameter (i.e., isthmus) about midway to the uterus.
Ovarian proper ligament: the strong fibrous band attaching the caudal pole of the ovaryto the cranial end of the uterus. (Fig. 13-8)
Uterus: The uterus is composed of two uterine horns, a singular body and a cervix.There are variations in the uterus among ungulate species. Make special note of the length of horns/body (a reflection of the degree of fusion of the paramesonephric ducts) and the internal irregularities associated with the cervical lumen (Fig. 13-11).
Recall that the broad ligament of the uterus connecting the uterus and ovary to the dorsal body wall, is made up of the mesometrium (supporting the uterus), mesovarium (supporting the ovary), and mesosalpinx (supporting the uterine tube).
Blood Supply: the (middle) uterine a. is the main arterial supply to the uterus of the COW, MARE, and SOW. The satellite vein of the uterine a. is small, and instead the ovarian v. is the main drainage vessel of the uterus.In RUMINANTS, the ovarian a. is intimately associated with the ovarian v. so that hormones draining from their production site in the uterus can diffuse back to the ovary via the ovarian a. (e.g., to result in lysis of the corpus luteum).
EQUINE
Ovary: The EQUINE ovary is quite large and indented at the ovulation fossa. This is the only site of ovulation in the mare.Note that the ovulation fossa is not present in the foal ovary but develops as the ovary matures. (The fossa is formed because the cortical tissue becomes restricted to the area of the ovulation fossa due to overgrowth and displacement by vascularized ‘medullary’ tissue.)
The ovaries can be palpated rectally to determine the pregnancy status of the female (although this is more commonly done in the COW). The uterus drapes over the pelvic brim (ventral and cranial edge of the pelvis) and this can be used as a landmark to follow the uterus to the ovaries (Fig. 13-9).
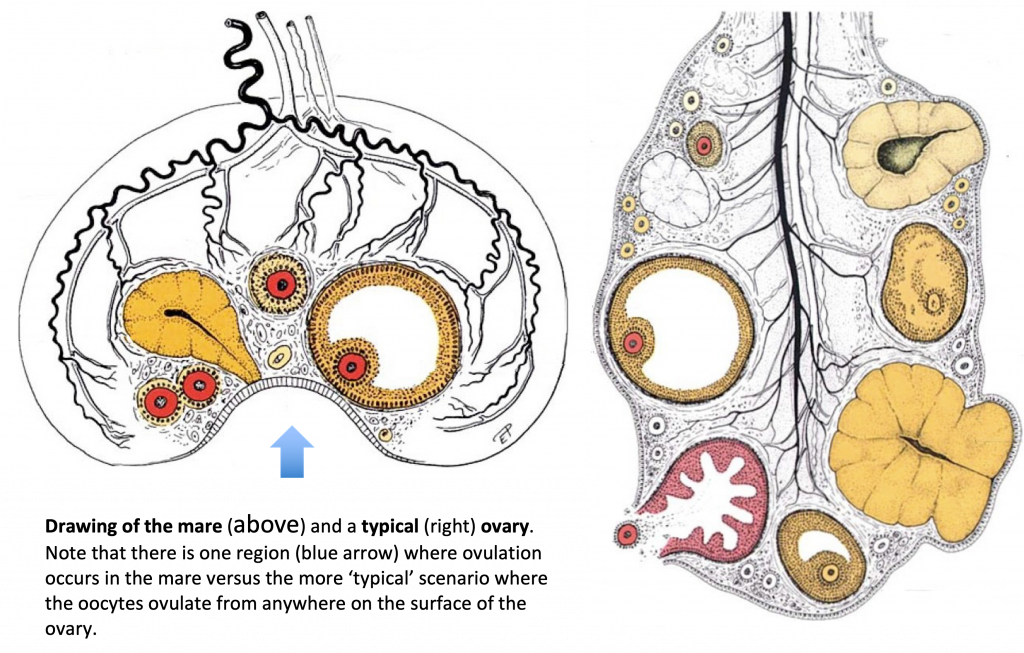
Figure – Ovulation fossa of mare on the left, typical ovary on the right.
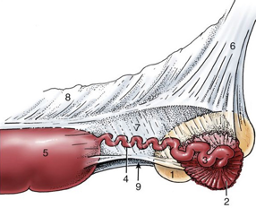
Figure 13-8 (Above) Mare right ovary, uterine tube and part of uterus (lateral view). 1, ovary; 2, infundibulum of tube; 3, ampulla of tube; 4, isthmus of tube; 5, uterine horn; 6, mesovarium; 7, mesosalpinx; 8, mesometrium; 9, arrow indicates entrance to ovarian bursa. (TVA 5-58)
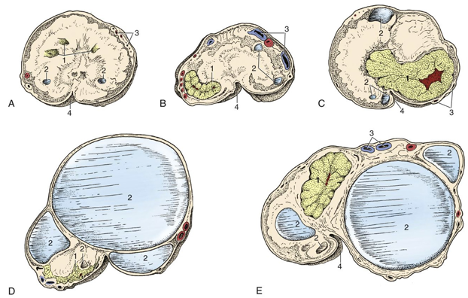
Figure 13B-3 (Above) Mare ovaries sectioned at various stages of follicular and corpus luteum development. A. Ovary with corpora lutea and small follicles. B, Ovary with developing corpus luteum. C, Ovary with fully developed corpus luteum. D Ovary with mature follicle. E, Ovary with follicles of various sizes and a rather large corpus luteum. Note how the corpus luteum of the mare does not protrude from the surface of the ovary as in other species. 1, corpora lutea; 2, follicles; 3, blood vessels; 4, ovulation fossa. (TVA 22-10)
Uterus: (Figure 13-9)Of all the common domestic animals, the MARE has the greatest degree of fusion of uterine horns to form a uterine body. The uterus is T-shaped with no distinctintercornual ligament and the cervix lacks transverse folds, therefore passage of an artificial insemination (AI) pipet is easier than in the COW (more details below in ruminant section). The cervix has an external os that opens into the vagina, which joins the exit of urinary system at the vestibule.
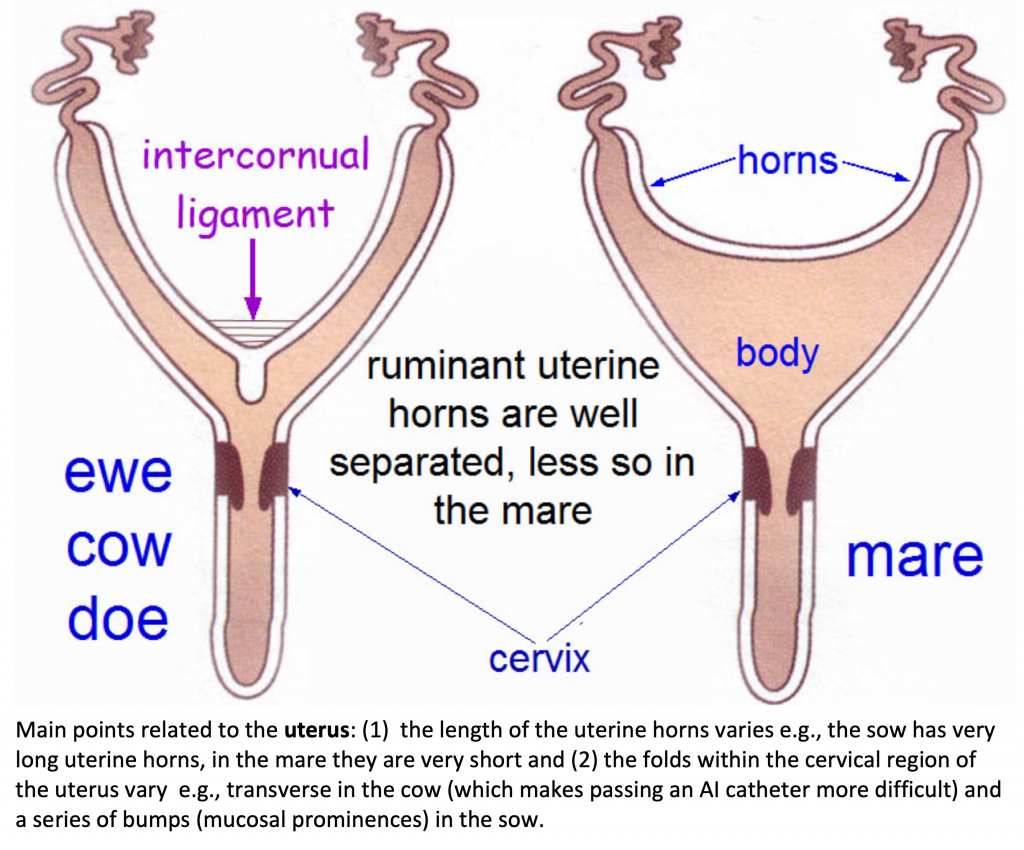
Clitoris and External Features: There are dorsal and ventral commissures of the vulva. At the ventral commissure of the vulva note the large MARE (glans) clitoris which resembles the glans penis of the male HORSE. The crura of the clitoris are deep to the mucosa of the vestibule.
The space surrounding the clitoris ventrally is the clitoral fossa. The clitoris is larger and more developed in the MARE than any other domestic animal. When the clitoris engorges and moves internal to external during estrus cycles, indicating sexually receptivity, this is sometimes called ‘winking.’
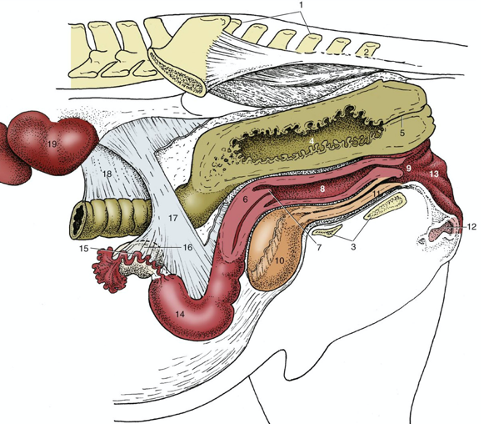
Figure 13-9 (Above) Mare caudal abdominal and pelvic organs; the organs have been sectioned in a paramedian plane with the pelvis. Because of the absence of the intestines, the ovaries hang much lower than they would in the intact animal. 1, sacrum; 2, Cd2; 3, floor of pelvis; 4, rectum; 5, anal canal; 6, cervix; 7, vaginal part of cervix; 8, vagina; 9, vestibule; 10, bladder; 11, urethra; 12, clitoris; 13, vulva; 14, left uterine horn; 15, uterine tube; 16, ovary; 17, broad ligament (largely cut away); 18, descending mesocolon; 19, left kidney. (TVA)
RUMINANT (bovine, caprine, ovine)
Ovary:The ovary of the COW is almond shaped with a smooth, free surface ventrally, and a broad hilus dorsally, which serves to anchor the organ and transmit its vascular supply.
The ovarian bursa communicates with the peritoneal cavity by a wide opening.
In the mature COW, the ovaries are positioned in the abdominal cavity, suspended by long mesenteric ligaments from the dorsolateral wall of the abdomen, just cranial to the pelvic inlet (Fig. 13B-4).
The ovaries can be examined for evidence of cyclic activity and/or pregnancy. Pregnant uteri will be associated with a functional corpus luteum; if found, the uterus should bepalpated to detect a fetus.
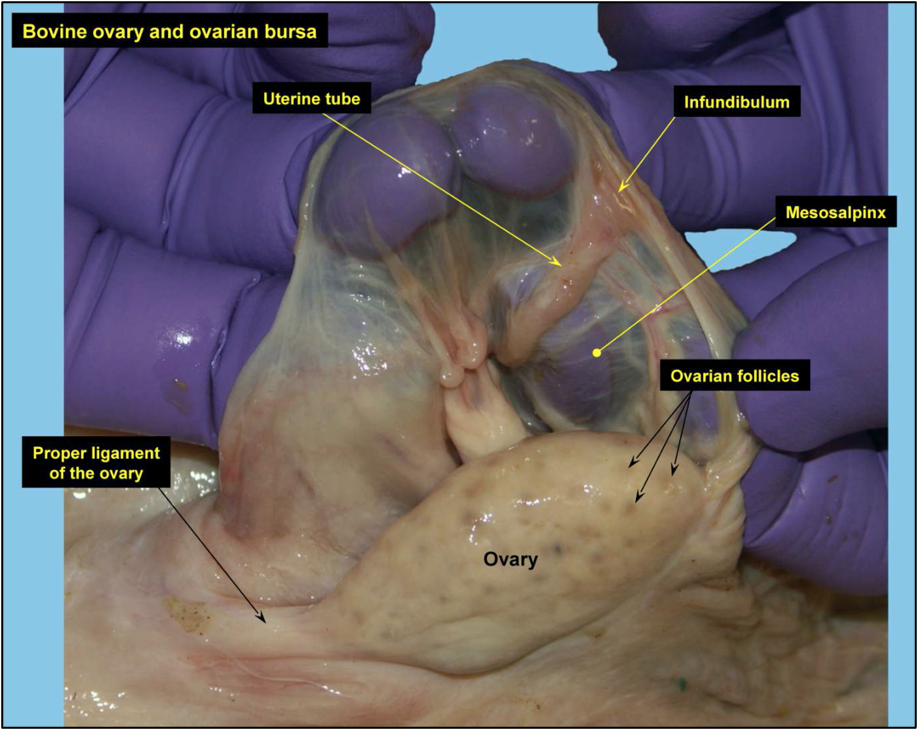
Uterus:In RUMINANTS, the uterine horns are long and coiled in a loose spiral. There is extensive fusion of the medial walls of the horns so that the body of the uterus is short.
Two intercornual ligaments (Figs. 13-10, 13-11, and 13B-4) connect the uterine horns(cornua) of the COW. In small RUMINANTS, only one intercornual ligament is present.Note that the dorsal intercornual ligament is an easily identifiable landmark in rectal palpation of the uterus in the COW. One can grab the intercornual ligaments to retract the uterus to palpate the ovaries and feel for different sized or shaped follicles on the surface.
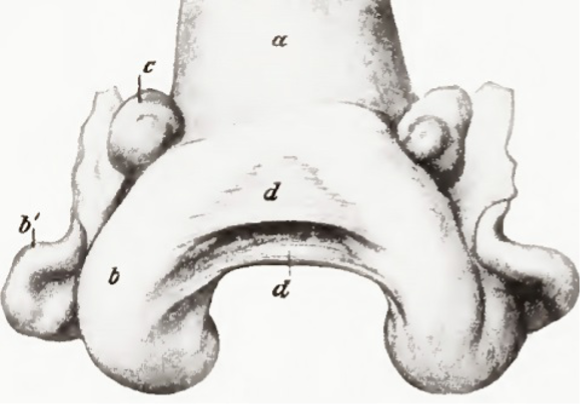
Figure 13-10 (Above) Cow uterus (contracted); dorsal view. a, Body of uterus; b,b’, horn of uterus; c, ovary; d, d, intercornual ligaments. (Sisson Grossman Fig. 535)
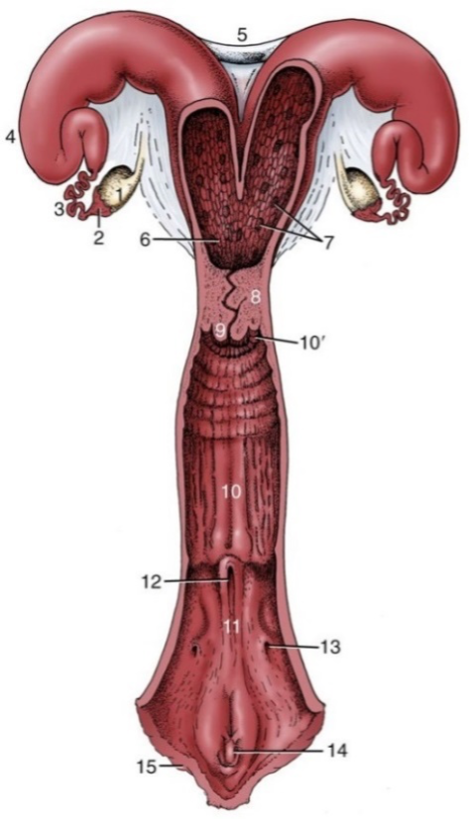
Figure 13-11 (Above) Cow reproductive tract, opened dorsally. 1, ovary; 2, infundibulum; 3, uterine tube; 4, horn of uterus; 5, intercornual ligaments; 6, body of uterus; 7, caruncles; 8, cervix; 9, vaginal part of cervix; 10, vagina; 10’, fornix; 11, vestibule; 12, external urethral opening; 13, opening of major vestibular gland; 14, clitoris; 15, vulva. (TVA 5-59)
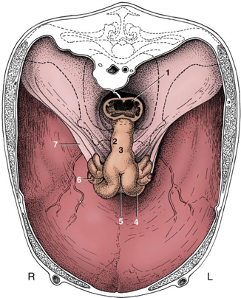
Figure 13B-4 (Above) Cow reproductive tract in situ, cranial view. The bony pelvis is indicated by broken lines. The uterus sags within this largely eviscerated abdomen. 1, Rectum; 2, cervix; 3, body of uterus; 4, left uterine horn; 5, intercornual ligament; 6, right ovary; 7, broad ligament. (TVA 29-15)
There is a short uterine body that leads to the uterine cervix. The cervix is markedly firmer to the touch than the uterine body.
RUMINANTs have caruncles on the uterine lining (i.e., the maternal side of the placenta). The fetal component of the placenta (i.e., cotyledons) adhere to the caruncles. Each caruncle/cotyledon interface is called a placentome. There are differences in some species related to the shape of the interface (e.g., sheep have concave shape).
Blood Supply: The BOVINE genital tract receives arterial blood from 3 pairs of bilateral arteries. Longitudinally distributing arteries connect these sources into a single system.The ovarian a. normally arises from the aorta and courses to the ovary. The ovarian a. sends branches to the uterine tube and to the cranial end of the uterus.
The ovarian a. is intimately associated with the ovarian v.. The ovarian a. is small compared with the vein and has a tortuous path on the vein in RUMINANTS but notin the MARE.
The major arterial supply to the uterus is from the uterine a., a branch of the internal iliac a. This artery reaches the uterine horn cranial to the uterine body and sends branches cranially to join the terminal distribution of the uterine branch of the ovarian a. and caudally to join with branches of the vaginal a.
The uterine a. is palpable rectally. Changes in its size are diagnostic of the stage of pregnancy the animal is in.
Within the cervix, there are transverse folds of the cervix that make passage of an artificial insemination (AI) pipet difficult. The external os of the cervix is the distal exit/opening of the cervix.
Clitoris and External Features:Urine exits the urinary bladder through the urethra via the urethral orifice (which empties into the vestibule). Caudal (and dorsal) to this point the vagina opens into the vestibule.
The urethral orifice opens into the suburethral diverticulum (Fig. 13B-5). This diverticulum is important because urinary catheters are often misdirected into the diverticulum.
The clitoris of the COW is small and lies near the ventral commissure of the vulva.
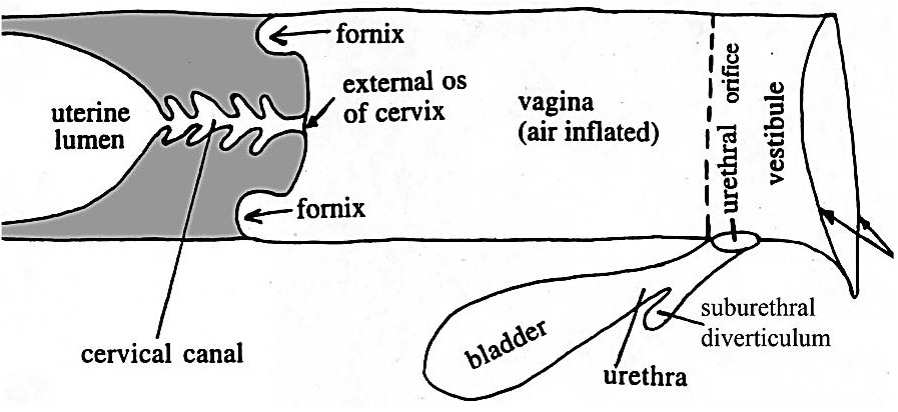
Figure 13B-5 (Above) Schematic illustration of caudal portion of a bovine female genital tract to show the location of the suburethral diverticulum. Arrows on the right = lips of vulva.
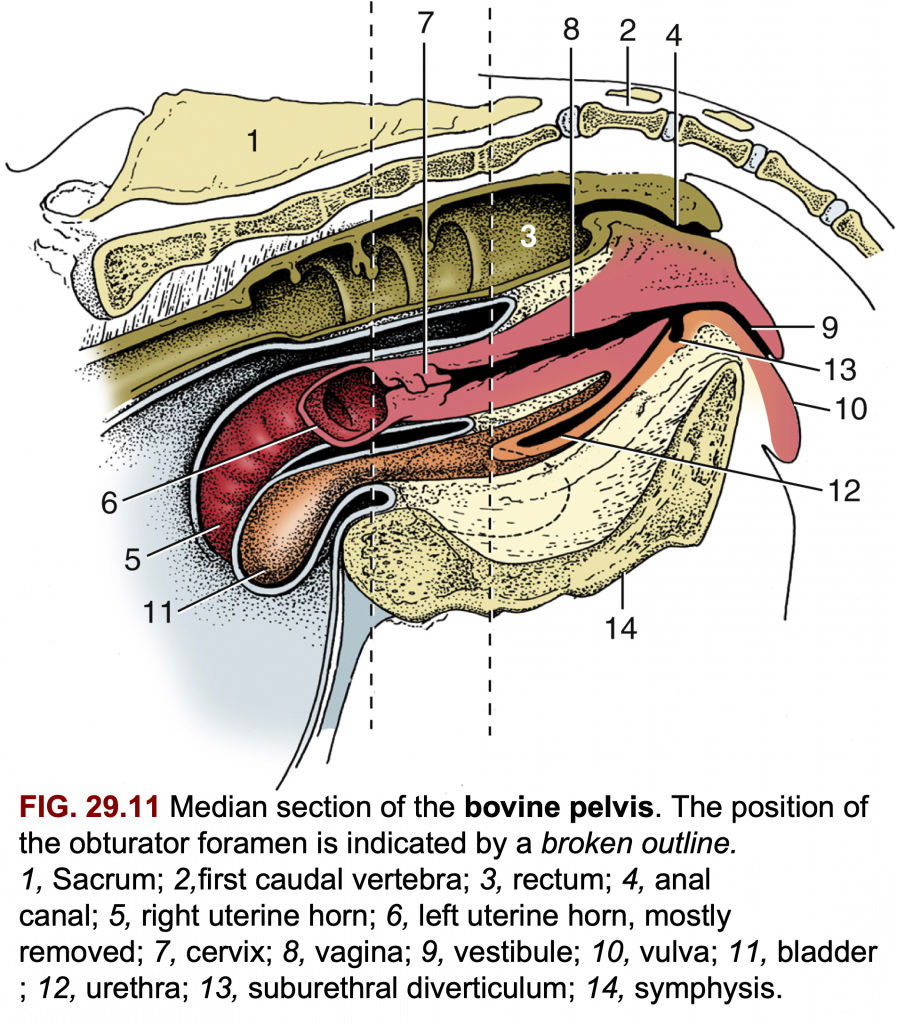
PORCINE
Ovary:The ovary of the SOW has an irregular surface due to its numerous follicles.
Uterus:In the SOW, the uterine horns are long, as is the cervix. However, the uterine body is quite short.
In the cervix of the SOW there are mucosal prominences (Fig. 13B-6), rather than transverse folds as seen in the cervix of the COW.
The SOW has a suburethral diverticulum, similar to the COW (Fig. 13B-7), so there can be difficulty with urinary catheters as well.
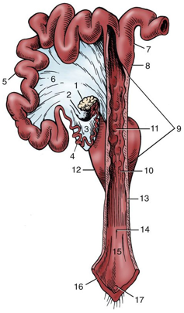
Figure 13B-6 (Above) Sow reproductive tract opened dorsally in part; the right uterine horn and ovary are not shown. 1, left ovary; 2, ovarian bursa; 3, mesosalpinx; 4, uterine tube; 5, uterine horn; 6, broad ligament; 7, parallel segments of uterine horns; 8, body of uterus; 9, cervix; 10, external uterine orifice; 11, mucosal prominences; 12, bladder, 13, vagina; 14, external urethral orifice; 15, vestibules; 16, vulva; 17, glans of clitoris. (TVA Fig 35-3)
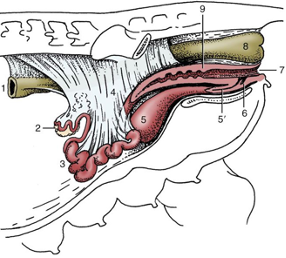
A9.7 Describe/identify the structures and connective tissue support of the bovine mammary glands (udder); describe the progressive changes in venous drainage of the udder with lactation.The BOVINE udder consists of four mammary glands (usually referred to as ‘quarters’). Each quarter has one teat.
Note the thick medial suspensory ligament of the udder which is yellow and elastic. It is derived from the tunica flava abdominis and attaches to the prepubic tendon. This paired midline structure completely separates the right and left parts of the mammary gland and makes surgical amputation of half of the udder possible.
The medial ligament is elastic and stretches more than the collagenous lateral laminae. Thus, the medial part of the distended udder sags most markedly and the teats point laterally due to the stronger (less elastic) pull of the lateral laminae.
The lateral laminae are more difficult to demonstrate. They extend from the subpelvic tendon, and medial femoral fascia, and penetrate the udder at various levels.
In EQUINE, the udder is small in the MARE. It consists of a pair of mammary glands, each with one teat.Note that in the EQUINE, there are two papillary ducts on each teat that drain separate lactiferous sinuses (i.e., there are two separate duct systems that drain into each teat). Hence, each teat also has two teat orifices.

Figure 13-12 (Above) Transverse section of the abdominal floor and cranial quarters of the bovine udder. 1, external abdominal oblique; 2, internal abdominal oblique; 3, rectus abdominis; 4, peritoneum; 5, linea alba; 6, lymph vessel; 7, external pudendal vein; 8, external pudendal (mammary) artery; 9, medial suspensory ligament; 10, lactiferous sinus; 11, papillary duct; 12, lateral laminae of suspensory apparatus. (Modified from TVA Figure 29-39).
Teats (Fig. 13-13)Sometimes there are supernumerary teats (any teats in excess of the normal number).
Features of normal teats: Teat orifice: the external opening of the teat.
Papillary duct (aka ‘teat canal’ or ‘streak canal’): the passage connecting the teat sinus with the teat orifice. The papillary duct has a tough lining and is thrown into folds by a sphincter muscle. Tight closure of the teat canal is essential to prevent entry of ascending organisms that could lead to the development of mastitis. The lining of the teat canal is stratified squamous tissue which is said to have antibacterial properties.
Teat sinus: the lumen of the teat.
Gland sinus: dorsal to the teat sinus and is separated from it by an inconstant annular fold at the base of the teat (internal only).
Lactiferous sinus: the collective term for the gland sinus and the teat sinus combined. The lactiferous sinus is only divided in the RUMINANTS due to the large size of their teats.
The fore and hind quarter of the udder cannot be distinctly separated. The glandsystems are, however, completely separate. Note that the hind quarters are considerably larger than the fore quarters.
Lactiferous ducts: the ducts which empty into the lactiferous sinus.
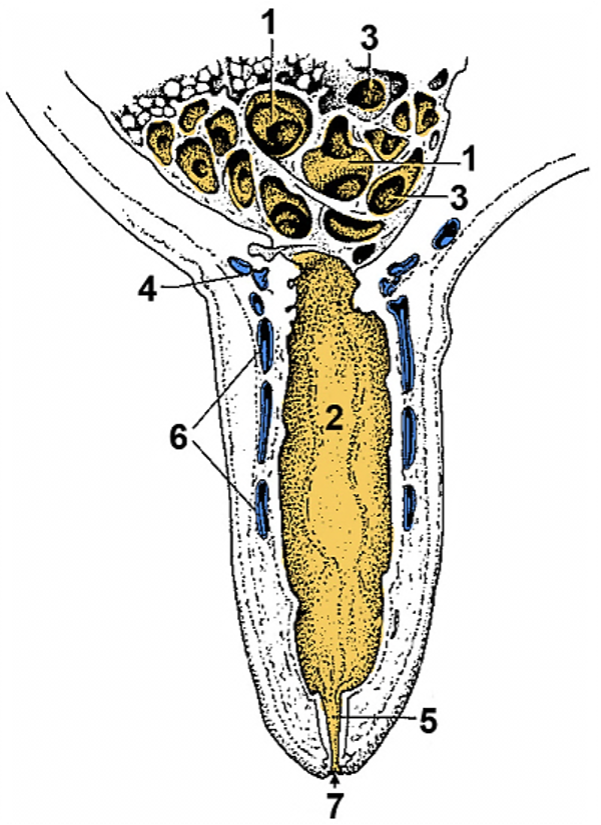
Figure 13-13 (Above) Section of a cow’s teat and lactiferous sinus. 1, 2, Lactiferous sinus = gland sinus (1) and teat sinus (2); 3, openings of lactiferous ducts into the lactiferous sinus; 4, submucosal venous ring; 5, papillary duct; 6, venous plexus in teat wall; 7, teat orifice. (Modified from TVA 3rd ed., Fig. 31-5
Blood Supply of the Udder (Figures 13B-8, 29-45, 29-44)The external pudendal a. and v. are on the caudolateral edge of the mammary gland. The external pudendal vv. course along the dorsal edge of the gland as they form a venous ring (i.e., around the base of the udder).
From the cranial part of the venous ring, in lactating cows, the paired subcutaneous abdominal vv. (aka ‘milk veins’) extend cranially, to the so called “milk well”, where they penetrate the body wall and join the internal thoracic vv. (Note, these internal thoracic vv. are running parallel to the internal thoracic aa. along the internal aspect of the sternum).
The subcutaneous abdominal v. hypertrophies with each lactation so that it is readily visible in mature individuals. (This vein is the result of the enlargement of the cranialand caudal superficial epigastric vv. and their anastomotic connection.)The PRIMARY venous drainage of the udder is through the external pudendal v., to the external iliac v., and then the caudal vena cava.
The SECONDARY venous drainage (external pudendal v., to subcutaneous abdominal v., to internal thoracic v.) is poorly developed in heifers and can result in udder edema (caked udder) in these individuals, due to insufficient venous drainage.
Reminder: typical venous drainage of the abdominal wall, non lactating or young animals:Cranial superficial epigastric vv. > internal thoracic vv. > cranial vena cava
Caudal superficial epigastric vv. > external pudendal vv. > external iliac vv. > caudal vena cava
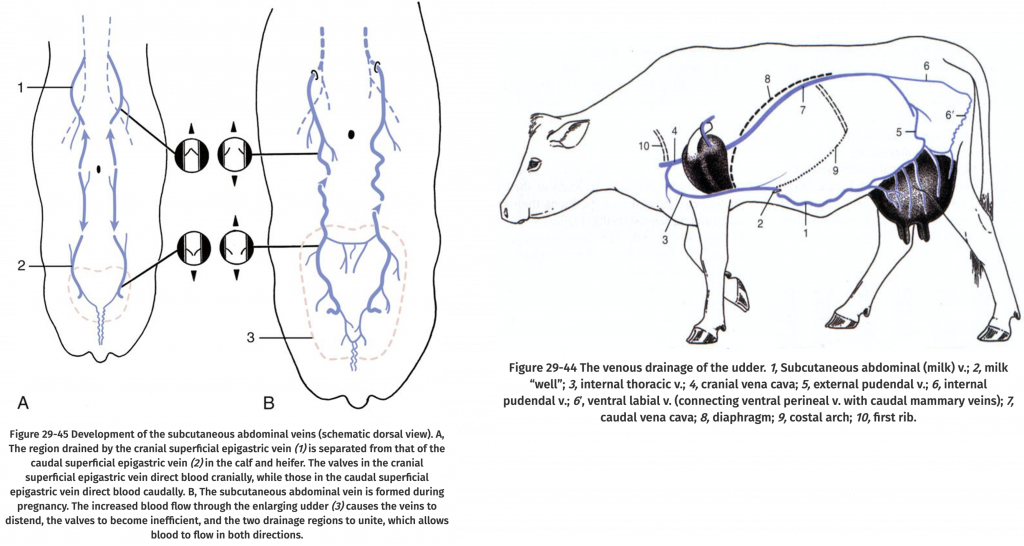
Figure (Above, left) Differences in subcutaneous abdominal veins in a calf/heifer versus a pregnant cow. (Above, right) Path of venous drainage in lactating cow.
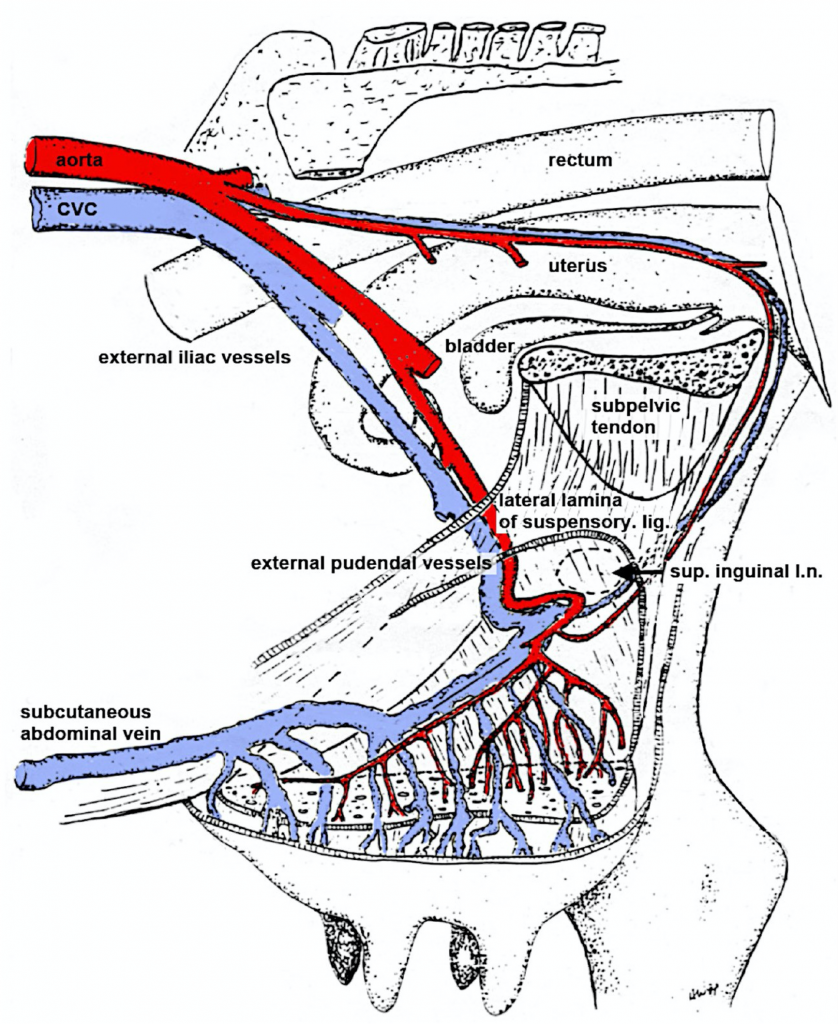
Figure 13B-8 (Above) Bovine udder, left lateral view (left limb removed). The subcutaneous abdominal vein represents the well-developed superficial epigastric veins. (Modified from original drawing by Dr. Alvin Weber).
Lymph Nodes (Figure 29-46)Note that the BOVINE has large paired mammary lymph nodes which lie near the caudal edge of the base of the udder. These nodes may be greatly enlarged in cattle with mastitis. They are actually superficial inguinal lymph nodes, and drain via the inguinal canal to the deep inguinal lymph nodes.
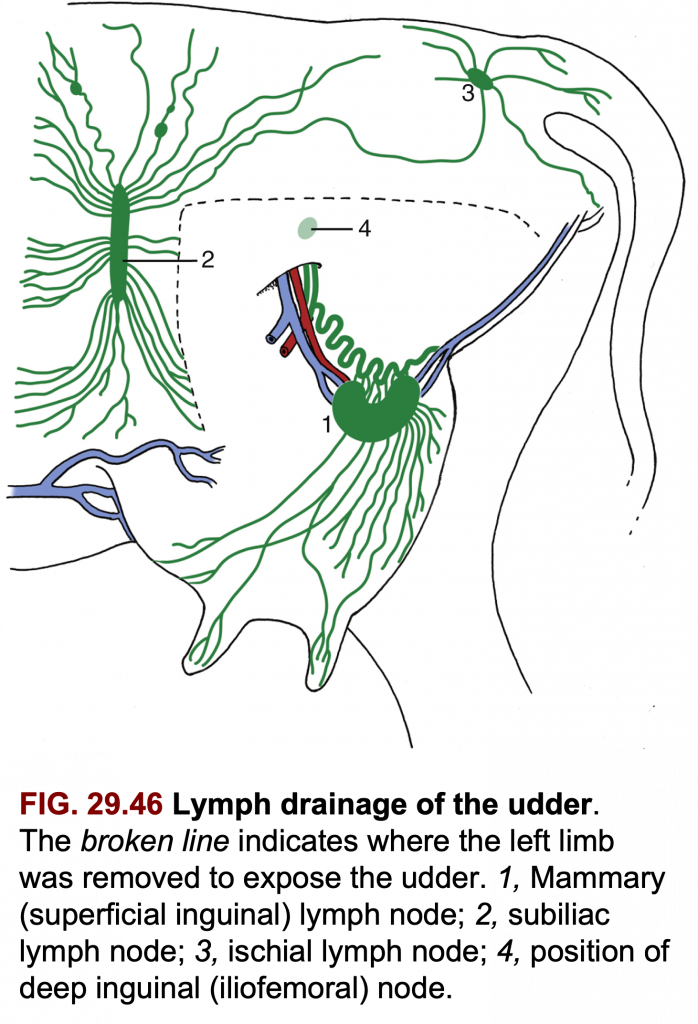
https://pressbooks.umn.edu/largeanimalanatomy/chapter/pelvis-genitalia/
CiteSeerX
[PDF] Classification of Developing Oocytes, Ovarian Development and Seasonal Variation in Rana tigerinaVisit
Images may be subject to copyright. Learn More
Comparative Histologic Evaluation of Vertebrate Ovaries
July 2018
Galician Medical Journal 25(2)
DOI:10.21802/gmj.2018.2.6
https://www.researchgate.net/publication/326106416_Comparative_Histologic_Evaluation_of_Vertebrate_Ovaries
Fallopian tubes
Author: Charlotte O'Leary BSc, MBChB • Reviewer: Jerome Goffin
Last reviewed: July 04, 2022
Reading time: 10 minutes
:background_color(FFFFFF):format(jpeg)/images/library/14050/BFN3R4Wa8jdbMlf7a1pf0Q_Tuba_uterina_01.png)
The fallopian tubes are important structures in the female reproductive tract, which connect the peritoneal cavity with the uterine cavity. They provide a site for fertilisation and are involved in the transport of the ovum from the ovaries to the body of the uterus. The fallopian tubes are also referred to as the uterine tubes or the oviducts.
This article will talk about the anatomy, histology, embryology and function of the fallopian tubes followed by any relevant clinical pathology.
Key facts about the fallopian tubesTable quizLocation Within the mesosalpinx, projecting from the superior body of the uterus
Parts Intramural, isthmus, ampulla, infundibulum (containing fimbriae)
Arterial supply Uterine and ovarian arteries
Venous drainage Uterine and pampiniform plexuses
Innervation Spinal segments T10-L2, pelvic splanchnic nerves, vagus nerve
Function Transport the ovum from the ovary to uterus
Location for fertilization
ContentsAnatomyLocation and trajectory
Parts
Arterial supply
Venous drainage
Lymphatic drainage
Innervation
Histology
Embryology
Function
Clinical notesSalpingitis
Endometriosis
Ligation
Ectopic tubal pregnancy
Sources
+ Show all
Recommended video: Uterus and ovaries
Structure of the vagina, uterus, fallopian tubes and ovaries.
Anatomy
Location and trajectory
The two fallopian tubes are about 10 cm long and project from the superior body of the uterus. They are located within the mesosalpinx, a component of the broad ligament of the uterus, and open medially at the superior angle of the uterus. The fallopian tubes extend in a superolateral direction, pass superior and anterior to the ovaries, and open into the peritoneal cavity lateral to them.
:watermark(/images/watermark_5000_10percent.png,0,0,0):watermark(/images/logo_url.png,-10,-10,0):format(jpeg)/images/overview_image/480/MANYFOgzoGYGWbGEabJTg_uterus-and-ovaries_english.jpg)
Uterus and ovaries
Parts
The fallopian tubes consist of four main parts, from medial to lateral:Intramural (interstitial) part, which is located within the myometrium of the uterus, is 1 cm long and 0.7 mm wide.
The isthmus, which is a lateral continuation of the intramural part. It is a rounded, muscular part of the fallopian tube. It is 3 cm long and between 1 and 5 mm wide.
The ampulla, which is longest part of the tube. It has a diameter of 1 cm at its widest point and is 5 cm long. It has a thin wall, a folded luminal surface and fertilisation usually takes place within its lumen.
The infundibulum, which is the distal end of the tube. It is funnel shaped and opens into the peritoneal cavity at the abdominal ostium. Finger like mucosal projections are attached to the distal end of the infundibulum and are referred to as fimbriae. These fimbriae are 1 mm wide and project over the medial surface of the ovaries. The longest of the fimbriae, the ovarian fimbria, attaches to the superior aspect of each ovary.
Why not try revising the parts of the fallopian tube with flashcards? Here's how you can make your own!
Arterial supply
:watermark(/images/watermark_only_sm.png,0,0,0):watermark(/images/logo_url_sm.png,-10,-10,0):format(jpeg)/images/anatomy_term/left-uterine-artery/kiYv1i9lei2rLuGvp3otGA_A._uterina_sinister_02.png)
:watermark(/images/watermark_only_sm.png,0,0,0):watermark(/images/logo_url_sm.png,-10,-10,0):format(jpeg)/images/anatomy_term/ovarian-vein-2/FXBTW6THXnpkaPUj8Kq3g_A._ovarii_01.png)
Left uterine artery
Arteria uterina sinistra
1/4
The arterial supply of the fallopian tubes involves both the uterine and ovarian arteries. The uterine artery supplies the medial two-thirds of the tube, whilst the lateral third is supplied by the ovarian artery.
Venous drainage
The uterine plexus drains the medial two-thirds of the fallopian tubes into the internal iliac veinwhereas the pampiniform plexus drains the lateral third. The pampiniform plexus drains into the ovarian veins, which in turn drain into the renal vein on the left and the inferior vena cavaon the right.
Lymphatic drainage
Lymph is also drained by both ovarian and uterine vessels, which drain into the para-aortic and internal iliac lymph nodes, respectively.
Innervation
The fallopian tubes are innervated by both the sympathetic and parasympathetic nervous systems. The sympathetic nerves arise from the spinal segments T10-L2. The parasympathetic nerves that supply the medial half of the tube are derived from the pelvic splanchnic nerves, whilst the fibres supplying the lateral half of the tube are derived from the vagus nerve.
:format(jpeg)/images/sequence/uterus-and-ovaries/KHh79ZeLHzef6DDjHePkfw_Uterus.png)
Structures of the uterus and ovaries seen with uterus straightened (33 structures).START QUIZ
108
Basic structure identification questions
33
Advanced structure identification questions
36
Exam questions (Question bank)
Histology
The walls of the fallopian tubes consist of three main layers:the mucosa
the muscularis
the serosa
The mucosa is comprised of longitudinal folds, more pronounced at the infundibulum, and is lined by a single layer of tall, columnar epithelium. There are three types of columnar cells within the epithelium: ciliated, non-ciliated secretory and intercalated cells. The ciliated cells are more predominant in the distal portion of the tubes and develop more cilia in the first half of the menstrual cycle. The wave like movement of the cilia aids in the movement of the ovum throughout the fallopian tubes. The longer non-ciliated secretory cells are more active during ovulation and unlike the ciliated cells, are more predominant in the proximal portion of the tubes. These cells secrete a fluid that is propelled with the ovum towards the uterus, by the cilia. The secretion provides a nutrient for the fertilised ovum and also aids in capacitation, a maturation step, of the spermatozoa. Post menopause, the epithelium decreases in height due to a reduction in the number of ciliated cells.
:background_color(FFFFFF):format(jpeg)/images/library/14049/uIZm0nOswiX0wvdecd63TA_Fallopian_tube.png)
The muscularis is arranged into two layers: an inner circular layer and an outer longitudinal layer. Innervation of these layers results in peristaltic contractions of the fallopian tubes, which assist in propulsion of the fertilised ovum.
Embryology
The fallopian tubes develop from the paramesonephric or Müllerian ducts. These ducts are derived from the mesoderm, the middle layer of one of the three primary germ layers in the embryo. The other two layers are the ectoderm and the endoderm. The tubes are derived from the superior vertical and middle horizontal aspects of the duct and undergo elongation and coiling to form the fully developed fallopian tubes.
Function
The fallopian tubes are involved in the transport of the ovum from the ovary to the uterus. This is aided by the peristaltic contractions of the muscular layers of the tubes and by the wave-like movement of the ciliated cells. During ovulation, the fimbriae swell which aids the movement of the released oocyte from the ovary to the fallopian tubes. The spermatozoa travel within the tubes towards the ovum and fertilisation usually occurs within the ampulla. Once fertilisation takes place, the tubes also provide nourishment for the fertilised ovum.
For more details about the anatomy of the fallopian tubes, take a look below:
:format(jpeg)/images/study_unit/uterus-and-ovaries/KHh79ZeLHzef6DDjHePkfw_Uterus.png)
Clinical notes
Salpingitis
Salpingitis or inflammation of the fallopian tubes is the most common pathology affecting the tubes and is usually part of a pelvic inflammatory disease affecting the uterus, the tubes and the ovaries. It usually results from a bacterial infection, leading to scarring of the tubes and subsequent tubal ectopic pregnancy. Obstruction of the fallopian tubes can be determined by hysterosalpingography or a hysteroscopy. A hysterosalpingography involves injecting a contrast material into the uterus and the fallopian tubes, whilst an X-ray beam is projected over these structures. Examination of the fallopian tubes with a hysteroscope, an endoscopic instrument, is referred to as a hysteroscopy.
Endometriosis
Endometriosis, defined as the presence of endometrial tissue outside the uterine cavity, is sometimes believed to be due to a backward flow of tissue shedded during menses through the fallopian tubes into the pelvis.
Ligation
Ligation, or tying, of the fallopian tubes is an effective surgical method of birth control. This prevents fertilisation of the oocyte, which then degenerate and become absorbed. There are two main types of ligation: abdominal tubal ligation and laparoscopic tubal ligation. Abdominal tubal ligation involves a suprapubic incision at the pubic hairline. Laparoscopic tubal ligation, on the other hand, involves insertion of a laparoscope through a small incision near the umbilicus.
Ectopic tubal pregnancy
When a fertilised ovum implants in the mucosa of the fallopian tubes this is referred to as an ectopic tubal pregnancy. This may occur due to obstruction of the fallopian tubes by pus, referred to as pyosalpinx. If not detected early, ectopic tubal pregnancy usually results in a tubal abortion, a rupture of the fallopian tube, during the first 8 weeks of gestation. This can cause haemorrhage into the peritoneal cavity, which can spread into the rectouterine pouch or into the uterovesical pouch. This can lead to inflammation of the parietal peritoneum, with the resulting pain referred to the right lower quadrant of the abdomen. A tubal abortion can sometimes be misdiagnosed as acute appendicitis, as it causes inflammation of the peritoneum in the same area. Haemorrhage from a tubal abortion can also irritate the subdiaphragmatic peritoneum, which can cause referred pain to the shoulder region via the phrenic nerve.
Sources
All content published on Kenhub is reviewed by medical and anatomy experts. The information we provide is grounded on academic literature and peer-reviewed research. Kenhub does not provide medical advice. You can learn more about our content creation and review standards by reading our content quality guidelines.
References:B. Young, J.S. Lowe, A. Stevens, J.W. Heath: Wheater’s Functional Histology: A Text and Colour Atlas, 5th Edition, Churchill Livingstone (2006), p. 368.
K.L. Moore, A.F. Dalley, A.M.R. Agur: Clinically Oriented Anatomy, 5th Edition, Lippincott Williams & Wilkins (2006), p. 424, 427.
R. L. Drake, A. Wayne, A.W.M. Mitchell: Gray’s Anatomy For Students, 2nd Edition, Churchill Livingstone (2010), 633-6.
R.S. Snell: Clinical Anatomy by Regions, 8th Edition, Lippincott Williams & Wilkins (2007), p. 363-4. • S. Standring: Gray’s Anatomy The Anatomical Basis Of Clinical Practice, 40th Edition, Elsevier Health Sciences UK (2008), p.2364-6.
Illustrators:Fallopian tubes (posterior view) - Samantha Zimmerman
Fallopian tubes (histological slide) - Smart In Media
Uterus and ovaries - Samantha Zimmerman
https://www.kenhub.com/en/library/anatomy/fallopian-tubes
The Fallopian tubes, also known as uterine tubes, salpinges (singular salpinx), or oviducts, are paired tubes that stretch from the ovaries to the uterus, in the human female reproductive system. In other mammals they are called oviducts.
Each tube is approximately 10cm in length and has five parts: the intramural part, isthmus, ampulla, infundibulum, and fimbria. Each tube has two openings: one communicates with the lateral angle of the uterine cavity and thus is called the uterine cavity; the other is called the pelvic opening or abdominal ostium, and lies on the lateral end of the tube.
A fertilized egg passes through the Fallopian tubes from the ovaries to the uterus, facilitates fertilization, an survival of zygote through its secretion. The Fallopian tubes are lined with simple columnar epithelium with hairlike extensions called cilia which carry the fertilized egg.
The name comes from the Catholic priest and anatomist Gabriele Falloppio, for whom other anatomical structures are also named.
Fallopian tube

Uterus and fallopian tubes

Cross-section of Fallopian tube, stained and viewed under microscope
Details
System Reproductive system
Artery tubal branches of ovarian artery, tubal branch of uterine artery via mesosalpinx
Lymph lumbar lymph nodes
Identifiers
Latin Tuba uterina
Greek Salpinx
MeSH D005187
TA98 A09.1.02.001
TA2 3486
FMA 18245
Anatomical terminology
Structure[edit]
The Fallopian tube is composed of four parts. These are, described from near the ovaries to inward near the uterus, the infundibulum with its associated fimbriae near the ovary, the ampulla that represents the major portion of the lateral tube, the isthmus, which is the narrower part of the tube that links to the uterus, and the interstitial (or intramural) part, the narrowest part of the uterine tube, that crosses the muscles of the uterine. The average length of a fallopian tube is 11–12 centimeters (4.3–4.7 in).[1] In other mammalsthe Fallopian tube is called the oviduct which may also be used in reference to the Fallopian tube in the human.[2][3]
Parts[edit]
The uterus opens into the Fallopian tube at the proximal tubal opening (also called the proximal ostium or os[4]), after the uterotubal junction, and accessible via hysteroscopy. Occlusion at this opening is referred to as proximal tubal occlusion. From here, there are three named parts of the Fallopian tube; the isthmus, the ampulla, and the infundibulum. The isthmus sits next to the opening of the Fallopian tube into the uterus. It connects to the ampulla (Latin: flask), which curves over the ovary and is the most common site of human fertilization.
The ampulla connects with the infundibulum, which rests above the ovaries, and ends at the distal tubal opening (or abdominal ostium)[5] into the abdominal cavitywhere, in ovulation, the oocyte enters the Fallopian tube. The opening is surrounded by fimbriae, which help in the collection of the oocyte. Occlusion of this opening is referred to as distal tubal occlusion. The fimbriae (singular fimbria) is a fringe of tissue around the ostium of the Fallopian tube, in the direction of the ovary. Of all fimbriae, one fimbria is long enough to reach the ovary. It is called fimbria ovarica.[6][7]
An ovary is not directly connected to its adjacent Fallopian tube. When ovulation is about to occur, the sex hormones activate the fimbriae, causing them to swell with blood and hit the ovary in a gentle, sweeping motion. An oocyte is released from the ovary into the peritoneal cavity and the cilia of the fimbriae sweep the ovum into the Fallopian tube.
Microanatomy[edit]
When viewed under the microscope, the Fallopian tube has four to five layers (depending on the classification system used). From outer to inner, these are the serosa, subserosa, muscularis, submucosa and innermost mucosa with lamina propria, and epithelium. The serosa is derived from the visceral peritoneum. The subserosa is composed of loose adventitious tissue, blood vessels, lymphatics. The muscularis consist of outer longitudinal and inner circular smooth muscle coats. This layer is responsible for the rhythmic contraction, called peristalsis, of the Fallopian tubes.[7] The histological features of tube vary along its length. The mucosa of the ampulla contains an extensive array of complex folds, whereas the relatively narrow isthmus has a thick muscular coat and simple mucosal folds.[7]
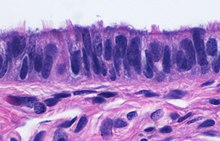
Histology of ciliated columnar epithelium of the Fallopian tube
The innermost layer of the tube is an epithelium composed of a single layer of column-shaped cells. The columnar cells have microscopic hair-like filaments called cilia throughout the tube, most numerous in the infundibulum and ampulla. Estrogenincreases the formation of cilia on these cells. Between the ciliated cells are peg cells, which contain apical granules and produce tubular fluid. This fluid contains nutrients for spermatozoa, oocytes, and zygotes. The secretions also promote capacitation of the sperm by removing glycoproteins and other molecules from the plasma membrane of the sperm. Progesterone increases the number of peg cells, while estrogen increases their height and secretory activity. Fluid flows through the tubes toward the ovaries, the opposite direction to the action of the cilia.
Development[edit]

Sketch of a human embryo from Gray's Anatomy; from eight and a half to nine weeks old, showing the mullerian (paramesonephric ducts), their position, and how they are becoming fused
Embryos develop a urogenital ridge that forms at their tail end and eventually forms the basis for the urinary system and reproductive tracts. Either side and to the front of this tract, around the sixth week develops a duct called the paramesonephric duct, also called the Müllerian duct.[8]: 530–531 A second duct, the mesonephric duct, develops adjacent to this. Both ducts become longer over the next two weeks, and the paramesonephric ducts around the eighth week cross to meet in the midline and fuse.[8]: 530–531 One duct then regresses, with this depending on whether the embryo is genetically female or male. In females, the paramesonephric duct remains, and eventually forms the female reproductive tract.[8]: 530–531 The portions of the paramesonephric duct which are more cranial—that is, further from the tail-end, end up forming the fallopian tubes.[8]: 530–531 In males, because of the presence of the Y sex chromosome, anti-Müllerian hormone is produced. This leads to the degeneration of the paramesonephric duct.[8]: 530–531
As the uterus develops, the part of the fallopian tubes closer to the uterus, the ampulla, becomes larger. Extensions from the fallopian tubes, the fimbriae, develop over time.
Apart from the presence of sex chromosomes, specific genes associated with the development of the fallopian tubes include the Wnt and Hox groups of genes, Lim1, Pax2, and Emx2.[8]: 530–531
Embryos have two pairs of ducts that will let gametes out of the body when they are adults; one pair (the Müllerian ducts) develops in females into the fallopian tubes, uterus, and vagina, while the other pair (the Wolffian ducts) develops in males into the epididymis and vas deferens.
The homologous organ in the male is the rudimentary appendix testis.[citation needed]
Function[edit]
Fertilization[edit]

After ovulation, the egg (oocyte) passes from the ovary (left) through the Fallopian tube to the uterus (right).
The fallopian tube allows the passage of an egg from the ovary to the uterus. When an oocyte is developing in an ovary, it is surrounded by a spherical collection of cells known as an ovarian follicle. Just before ovulation, the primary oocyte completes meiosis I to form the first polar body and a secondary oocyte which is arrested in metaphase of meiosis II.
At the time of ovulation in the menstrual cycle, the secondary oocyte is released from the ovary. The follicle and the ovary's wall rupture, allowing the secondary oocyte to escape. The secondary oocyte is caught by the fimbriated end of the fallopian tube and travels to the ampulla. Here, the egg is able to become fertilized with sperm. The ampulla is typically where the sperm are met and fertilization occurs; meiosis II is promptly completed. After fertilization, the ovum is now called a zygote and travels toward the uterus with the aid of the hairlike cilia and the activity of the muscle of the fallopian tube. The early embryo requires critical development in the fallopian tube.[2] After about five days, the new embryo enters the uterine cavity and, on about the sixth day, implants on the wall of the uterus.
The release of an oocyte does not alternate between the two ovaries and seems to be random. After removal of an ovary, the remaining one produces an egg every month.[9]
Clinical significance[edit]
Inflammation[edit]
Main article: Salpingitis
Salpingitis is inflammation of the fallopian tubes and may be found alone, or as part of pelvic inflammatory disease (PID). A thickening of the fallopian tube at its narrow portion, due to inflammation, is known as salpingitis isthmica nodosa. Like PID and endometriosis, it may lead to fallopian tube obstruction. Fallopian tube obstruction may be a cause of infertility or ectopic pregnancy.[10]
Cancer[edit]
Main article: Fallopian tube cancer
Fallopian tube cancer, which typically arises from the epithelial lining of the fallopian tube, has historically been considered to be a very rare malignancy. Recent evidence suggests it probably represents a significant portion of what has been classified as ovarian cancer in the past.[11] While tubal cancers may be misdiagnosed as ovarian cancer, it is of little consequence as the treatment of both ovarian and fallopian tube cancer is similar.[citation needed]
Implantation[edit]
Main article: Ectopic pregnancy

lmplantation sites resulting in normal or ectopic pregnancies
Occasionally the embryo implants into the fallopian tube instead of the uterus, creating an ectopic pregnancy, commonly known as a "tubal pregnancy".
Blockage or narrowing[edit]
If a blocked fallopian tube has affected fertility, addressing the blockage can usually increase the chances of becoming pregnant. There are a few different ways to do that, based on where the blockage is located and if it can easily be removed.[12][13]
While full testing of tubal functions in patients with infertility is not possible, testing of whether the tubes are open, called patency, is important as tubal obstruction is a major cause of infertility. A hysterosalpingogram, laparoscopy and dye, or hysterocontrast sonography will demonstrate whether the tubes are open. Tubal insufflation is a standard procedure for testing patency. During surgery, the condition of the tubes may be inspected and a dye such as methylene blue can be injected into the uterus and shown to pass through the tubes when the cervix is occluded. As tubal disease is often related to Chlamydia infection, testing for Chlamydia antibodies has become a cost-effective screening device for tubal pathology.[14]
Surgery[edit]

Example and location of some surgical procedures performed on the Fallopian tubes
The surgical removal of a fallopian tube is called a salpingectomy. To remove both tubes is a bilateral salpingectomy. An operation that combines the removal of a fallopian tube with the removal of at least one ovary is a salpingo-oophorectomy. An operation to remove a fallopian tube obstruction is called a tuboplasty.
Other[edit]
It is possible for the Fallopian tube to prolapse into the vaginal canal following a hysterectomy, if the vaginal cuff is open. Given the beefy-red appearance of the tubes, it is possible to mistake the tube for either a tumor or granulation tissue at the vaginal cuff.[15]
History[edit]
The Fallopian tubes are named after the 16th-century Italian anatomist Gabriele Falloppio, the first person to provide a detailed description of the tubes.[16][8]: 530 He thought they resembled trumpets, tube in Italian, which was misunderstood and became the English "tube".[17]
Though the name Fallopian tube is eponymous, it is often spelt with a lower case f from the assumption that the adjective fallopian has been absorbed into modern English as the de facto name for the structure.[citation needed]
Additional images[edit]

Numbered image showing parts of the Fallopian tubes and surrounding structures:
1. ovary
2. medial surface
3. lateral surface
4. free border
5. mesovarial margin
6. tubal extremity
7. uterine extremity
8. Fallopian tube
9. distal opening of the Fallopian tube
10. infundibulum of the Fallopian tube
11. fimbriae of the Fallopian tube
12. ovarian fimbria
13. ampulla of the Fallopian tube
14. isthmus of the Fallopian tube
15. uterine part of the Fallopian tube
16. proximal opening of the Fallopian tube

Female reproductive system:
1. Vulva: 2. labia majora, 3. labia minora, 4. vestibule
5. Clitoris: (with 6. glans and 7. body) 8. bulb of vestibule
9. Vagina: 10. hymen, 11. lumen, 12. wall, 13. fornix (lateral)
14. Uterus: Parts: 15. cervix, 16. body and 17. fundus, 18. orifices: external and internal, 19. cervical canal, 20. uterine cavity; Layers: 21. endometrium, 22. myometrium, and 23. perimetrium
24. Fallopian tube: 25. isthmus, 26. ampulla, 27. infundibulum, 28. fimbria (with 29. fimbria ovarica)
30. Ovary
31. Visceral pelvic peritoneum: 23. perimetrium, 32. broad ligament(with 33. mesosalpinx, 34. mesovarium, and 35. mesometrium)
Ligaments: 36. round, 37. ovarian, 38. suspensory of ovary
Blood vessels: 39. ovarian artery and vein, 40. uterine artery and veins, 41. vaginal artery and veins
Other: 42. ureter, 43. pelvic floor (levator ani), 44. femoral head, 45. hip bone, 46. internal iliac vessels (anterior branches), 47. external iliac vessels, 48. abdominal cavity

Image showing the right Fallopian tube (here labeled the uterine tube) seen from behind. The uterus, ovaries and right broad ligament are labeled.

Isthmus of the Fallopian tube seen arising from the uterus in a cadavericspecimen
See also[edit]
This article uses anatomical terminology.Menstrual cycle
Internal |
| ||||||||||||||||||||||||||
---|---|---|---|---|---|---|---|---|---|---|---|---|---|---|---|---|---|---|---|---|---|---|---|---|---|---|---|
External |
| ||||||||||||||||||||||||||
Other |
The paroophoron (of Johnson) consists of a few scattered rudimentary tubules, best seen in the child, situated in the broad ligament between the epoöphoron and the uterus.[1] Named for the Welsh anatomist David Johnson who originally described the structure at the University of Wales, Aberystwyth.
It is a remnant of the mesonephric tubules.[2]
Paroophoron | |
---|---|
![]() Broad ligament of adult, showing epoöphoron. (From Farre, after Kobelt.) a, a. Epoöphoron formed from the upper part of the Wolffian body. b. Remains of the uppermost tubes sometimes forming appendices. c. Middle set of tubes. d. Some lower atrophied tubes. e. Atrophied remains of the Wolffian duct. f. The terminal bulb or hydatid. h. The uterine tube, originally the duct of Müller. i. Appendix attached to the extremity. l. The ovary.[1] | |
Details | |
Precursor | Mesonephric tubules |
Identifiers | |
Latin | Paroophoron |
TA98 | A09.1.06.001 |
TA2 | 3544 |
FMA | 18692 |
Anatomical terminology |
https://en.wikipedia.org/wiki/Paroophoron
The urethral crest is an anatomical feature present in the urinary system of both males and females.
In males, the urethral crest is known as the crista urethralis masculinae, or the crista phallica, and is a longitudinal fold on the posterior wall of the urethra extending from the uvula of the bladder through the prostatic urethra.[1] It is from 15 to 17 mm. in length, and about 3 mm. in height, and contains muscular and erectile tissue. When distended, it may serve to prevent the passage of the semen backward into the bladder.[2]
In females, it is known as the crista urethralis femininae, and is a conspicuous longitudinal fold of mucosa on the posterior wall of the urethra.[3]
Additional images[edit]
Urethral crest | |
---|---|
![]() The male urethra laid open on its anterior (upper) surface. (Urethral crest labeled at upper right.) | |
![]() Dissection of prostate showing prostatic urethra and urethral crest on posterior wall | |
Details | |
Identifiers | |
Latin | crista urethralis urethrae masculinae, crista urethralis urethrae femininae |
TA98 | A09.2.03.004 A09.4.02.007 |
TA2 | 3430, 3446 |
FMA | 19718 |
Anatomical terminology |
Follicular fluid | |
---|---|
![]() Human ovum examined fresh in the liquor folliculi | |
Details | |
Identifiers | |
Latin | liquor folliculi |
MeSH | D015571 |
FMA | 18665 |
Anatomical terminology |
The larger ovarian follicles consist of an external fibrovascular coat, connected with the surrounding stroma of the ovaryby a network of blood vessels, and an internal coat, which consists of several layers of nucleated cells, called the membrana granulosa. It contains numerous granulosa cells.
At one part of the mature follicle the cells of the membrana granulosa are collected into a mass which projects into the cavity of the follicle. This is termed the discus proligerus.
Membrana granulosa | |
---|---|
![]() Section of vesicular ovarian follicle of cat. X 50. (Membrana granulosa labeled at upper left.) | |
Anatomical terminology |
https://en.wikipedia.org/wiki/Membrana_granulosa
The cumulus oophorus, (discus proligerus), is a cluster of cells that surround the oocyte both in the ovarian follicle and after ovulation. In the antral follicle, it may be regarded as an extension of the membrana granulosa. The innermost layer of these cells is the corona radiata.[1]
This layer of cells must be penetrated by spermatozoa for fertilization to occur.
Cumulus oophorus | |
---|---|
![]() Section of vesicular ovarian follicle of cat. X 50. (Discus proligerus labeled at lower left.) | |
Details | |
Identifiers | |
Latin | Cumulus oophorus Discus proliger |
FMA | 18659 |
Anatomical terminology |
Functions[edit]
Functions of the cumulus oophorus include coordination of follicular development and oocyte maturation.[2] Mechanisms of the latter include stimulation of amino acid transport and sterol biosynthesis and regulation of oocyte gene transcription.[2]
It also provides energy substrates for oocyte meiotic resumption and promotes glycolysis.[2]
Cumulus oophorus cells contribute heavily to the maturation and eventual fertilization of an oocyte. As a follicle grows in size and the antrum develops, more layers of cumulus oophorus cells accumulate around the oocyte to aid in the acrosome reaction and sperm penetration into the oocyte. The proximity between the cumulus oophorus cells and the oocyte favors bidirectional communication, which is vital for oocyte development.
Gene expression profiling[edit]
As a part of the process of in vitro fertilization, gene expression profiling of cumulus cells can be performed to estimate oocyte quality and the efficiency of an ovarian hyperstimulation protocol, and may indirectly predict oocyte aneuploidy, embryo development and pregnancy outcomes. Increased knowledge in these aspects is useful in, for example, embryo selection.[citation needed]
In gene expression profiling of cumulus cells, genes where increased expression is correlated with higher oocyte competence or better pregnancy outcomes, include: HAS2, GREM1 and PTGS2.
In contrast, genes where increased expression is correlated with lower oocyte competence or worse pregnancy outcomes include: BDNF, CCND2, CXCR4, GPX3, HSPB1, DVL3, DHCR7, CTNND1, TRIM28, STAR, AREG, CX43, PTGS2, SCD1 and SCD5.[citation needed]
https://en.wikipedia.org/wiki/Cumulus_oophorus
The corona radiata is the innermost layer of the cells of the cumulus oophorus and is directly adjacent to the zona pellucida, the inner protective glycoprotein layer of the ovum.[1] Cumulus oophorus are the cells surrounding corona radiata, and are the cells between corona radiata and follicular antrum. Its main purpose in many animals is to supply vital proteins to the cell.[citation needed] It is formed by follicle cells adhering to the oocyte before it leaves the ovarian follicle, and originates from the squamous granulosa cells present at the primordial stage of follicular development. The corona radiata is formed when the granulosa cells enlarge and become cuboidal, which occurs during the transition from the primordial to primary stage. These cuboidal granulosa cells, also known as the granulosa radiata, form more layers throughout the maturation process, and remain attached to the zona pellucida after the ovulation of the Graafian follicle. For fertilization to occur, sperm cells rely on hyaluronidase (an enzyme found in the acrosome of spermatozoa) to disperse the corona radiata from the zona pellucida of the secondary (ovulated) oocyte, thus permitting entry into the perivitelline space and allowing contact between the sperm cell and the nucleus of the oocyte.
References[edit]
- ^ Pansky, Ben (1982), "Chapter 12: Fertilization", Review of MEDICAL EMBRYOLOGY, LifeMap Discovery, archived from the original on August 8, 2018, retrieved December 31, 2018
Corona radiata | |
---|---|
![]() Human ovum examined fresh in the follicular fluid. The zona pellucida is seen as a thick clear girdle surrounded by the cells of the corona radiata. The egg itself shows a central granular deutoplasmic area and a peripheral clear layer, and encloses the germinal vesicle, in which is seen the germinal spot. | |
Anatomical terminology |
https://en.wikipedia.org/wiki/Corona_radiata_(embryology)
Ovaries |
| ||||
---|---|---|---|---|---|
Fallopian tubes |
https://en.wikipedia.org/wiki/Corona_radiata_(embryology)
The uterine horns are the points where the uterus and the fallopian tubes meet. It is also known as the Cornua of Uterus (the superolateral angle of the body project outwards at the junction of the body and the fundus). They are one of the points of attachment for the round ligament of uterus (the other being the mons pubis). The Cornua also provides attachment to the ligament of ovary, which is located posteroinferior to the fallopian tube; while the round ligament of uterus is located anteroinferior to the fallopian tube.
The uterine horns are far more prominent in other animals (such as cows[1] and cats[2]) than they are in humans. In the cat, implantation of the embryo occurs in one of the two uterine horns, not the body of the uterus itself.
Occasionally, if a fallopian tube does not connect, the uterine horn will fill with blood each month, and a minor one-day surgery will be performed to remove it. Often, people who are born with this have trouble getting pregnant as both ovariesare functional and either may ovulate. The spare egg, that cannot travel the fallopian tube, is absorbed into the body.
Uterine horns | |
---|---|
![]() Uterine horn labeled in upper right. | |
![]() Uterine horn not labeled, but visible. The round ligament is at the left, labeled as #1. It travels to the right, and attaches to the uterus at the center. The fallopian tube is unnumbered, but it is visible above the uterus, and travels downward to attach at a location near the round ligament. | |
Details | |
Identifiers | |
Latin | cornu uteri |
TA98 | A09.1.03.004 |
FMA | 77053 |
Anatomical terminology |
https://en.wikipedia.org/wiki/Uterine_horns
The stroma of the ovary is a unique type of connective tissue abundantly supplied with blood vessels, consisting for the most part of spindle-shaped stroma cells. These appear similar to fibroblasts. The stroma also contains ordinary connective tissue such as reticular fibers and collagen. Ovarian stroma differs from typical connective tissue in that it contains a high number of cells. The stoma cells are distributed in such a way that the tissue appears to be whorled. Stromal cells associated with maturing follicles may acquire endocrine function and secrete estrogens. The entire ovarian stroma is highly vascular.[1]
On the surface of the organ this tissue is much condensed, and forms a layer (tunica albuginea) composed of short connective-tissue fibers, with fusiform cells between them.
The stroma of the ovary may contain interstitial cells resembling those of the testis.
Stroma of ovary | |
---|---|
![]() Section of the ovary. 1. Outer covering. 1’. Attached border. 2. Central stroma. 3. Peripheral stroma. 4. Blood vessels. 5. Vesicular follicles in their earliest stage. 6, 7, 8. More advanced follicles. 9. An almost mature follicle. 9’. Follicle from which the ovum has escaped. 10. Corpus luteum. | |
![]() Section of the fold in the mesonephros of a chick embryo of the fourth day. (Stroma of ovary labeled at center left.) | |
Details | |
Identifiers | |
Latin | stroma ovarii |
Anatomical terminology |
https://en.wikipedia.org/wiki/Stroma_of_ovary
The tunica albuginea is a layer of condensed fibrous tissue on the surface of the ovary.
Structure[edit]
The tunica albuginea is composed of short connective tissue fibers. It is located immediately inside the surface epithelium(previously known as germinal epithelium) which is continuous with the peritoneum. It is non-vascularised.[1] It is thinner than the tunica albuginea of the testis, and its thickness varies across the ovary.[1]
Development[edit]
The tunica albuginea is formed late in prenatal development.[1] It buds off from mesonephric stroma.[1]
This article incorporates text in the public domain from page 1256 of the 20th edition of Gray's Anatomy (1918)
- ^ ab c d Hummitzsch, Katja; Irving-Rodgers, Helen F.; Schwartz, Jeff; Rodgers, Raymond J. (2019-01-01), Leung, Peter C. K.; Adashi, Eli Y. (eds.), "Chapter 4 - Development of the Mammalian Ovary and Follicles", The Ovary (Third Edition), Academic Press, pp. 71–82, ISBN 978-0-12-813209-8, retrieved 2021-02-03
https://en.wikipedia.org/wiki/Tunica_albuginea_(ovaries)
The ovarian surface epithelium, also called the germinal epithelium of Waldeyer,[1] or coelomic epithelium is a layer of simple squamous-to-cuboidal epithelial cells covering the ovary.[2]
The term germinal epithelium is a misnomer as it does not give rise to primary follicles.[3]
Composition[edit]
These cells are derived from the mesoderm during embryonic development and are closely related to the mesothelium of the peritoneum. The germinal epithelium gives the ovary a dull gray color as compared with the shining smoothness of the peritoneum; and the transition between the mesothelium of the peritoneum and the cuboidal cells which cover the ovary is usually marked by a line around the anterior border of the ovary.
Diseases[edit]
Ovarian surface epithelium can give rise to surface epithelial-stromal tumor.
References[edit]
This article incorporates text in the public domain from the 20th edition of Gray's Anatomy (1918)
- ^ Nishida T, Nishida N (2006). "Reinstatement of "germinal epithelium" of the ovary". Reprod Biol Endocrinol. 4: 42. doi:10.1186/1477-7827-4-42. PMC 1560142. PMID 16923182.
- ^ Auersperg, N.; Wong, A. S.; Choi, K. C.; Kang, S. K.; Leung, P. C. (2001). "Ovarian Surface Epithelium: Biology, Endocrinology, and Pathology". Endocrine Reviews. 22 (2): 255–288. doi:10.1210/edrv.22.2.0422. PMID 11294827. S2CID 11013148.
- ^ Ross M, Pawlina W (2011). Histology: A Text and Atlas (6th ed.). Lippincott Williams & Wilkins. p. 832. ISBN 978-0-7817-7200-6.
External links[edit]
- Histology image: 18403loa – Histology Learning System at Boston University
- Histology image: 83_07 at the University of Oklahoma Health Sciences Center
Germinal epithelium (female) | |
---|---|
![]() Section of the ovary. 1. Germinal epithelium. 2. Central stroma. 3. Peripheral stroma. 4. Bloodvessels. 5. Vesicular follicles in their earliest stage. 6, 7, 8. More advanced follicles. 9. An almost mature follicle. 9'. Follicle from which the ovum has escaped. 10. Corpus luteum. | |
Identifiers | |
FMA | 18629 |
Anatomical terminology |
This article is part of a series on |
Epithelia |
---|
Squamous epithelial cell |
Columnar epithelial cell |
Cuboidal epithelial cell |
Specialised epithelia |
Other |
https://en.wikipedia.org/wiki/Germinal_epithelium_(female)
The mesothelium is a membrane composed of simple squamous epithelial cells of mesodermal origin,[1] which forms the lining of several body cavities: the pleura (pleural cavity around the lungs), peritoneum (abdominopelvic cavity including the mesentery, omenta, falciform ligament and the perimetrium) and pericardium (around the heart).
Mesothelial tissue also surrounds the male testis (as the tunica vaginalis) and occasionally the spermatic cord (in a patent processus vaginalis). Mesothelium that covers the internal organs is called visceral mesothelium, while one that covers the surrounding body walls is called the parietal mesothelium. The mesothelium that secretes serous fluid as a main function is also known as a serosa.
Mesothelium | |
---|---|
![]() | |
![]() A layer of mesothelial cells grown in cell culture, featuring the typical "cobblestone" appearance | |
Details | |
Precursor | Somatopleuric mesenchyme |
Identifiers | |
Latin | Mesothelium |
TH | H2.00.02.0.02017, H3.04.08.0.00003 |
FMA | 14074 |
Anatomical terminology |
Structure[edit]
The mesothelium forms a monolayer of flattened squamous-like epithelial cells resting on a thin basement membranesupported by dense irregular connective tissue. Cuboidal mesothelial cells may be found at areas of injury, the milky spotsof the omentum, and the peritoneal side of the diaphragm overlaying the lymphatic lacunae. The luminal surface is covered with microvilli. The proteins and serosal fluid trapped by the microvilli provide a slippery surface for internal organs to slide past one another.
Function[edit]
The mesothelium is composed of an extensive monolayer of specialized cells (mesothelial cells) that line the body's serous cavities and internal organs. The main purpose of these cells is to produce a lubricating fluid that is released between layers,[2] providing a slippery, non-adhesive, and protective surface to facilitate intracoelomic movement.
The mesothelium is also implicated in the transport and movement of fluid and particulate matter across the serosal cavities, leukocyte migration in response to inflammatory mediators, synthesis of pro-inflammatory cytokines, growth factors, and extracellular matrix proteins to aid in serosal repair, and the release of factors to promote the disposition and clearance of fibrin (such as plasminogen). Mesothelial cells are capable of phagocytosis and are antigen-presenting cells. The secretion of glycosaminoglycans and lubricants may protect the body against infection and tumor dissemination.
Role in disease[edit]
- Mesothelioma: (cancer of the mesothelium) is a disease in which cells of the mesothelium become abnormal and divide without control or order. They can invade and damage nearby tissues and organs. Cancer cells can also metastasize (spread) from their original site to other parts of the body. Most cases of mesothelioma begin in the pleura or peritoneum. More than 90% of mesothelioma cases are linked to asbestos exposure.
- Mesothelial hyperplasia
- Intra-abdominal adhesions: Normally, the mesothelium secretes plasminogen, which removes fibrin deposits. During surgical procedures, the mesothelium may be damaged. Its fibrinolytic capacity becomes insufficient and fibrin accumulates, causing fibrous adhesions between opposing surfaces. These adhesions cause intestinal obstruction and female infertility if it occurs in the abdomen, and may impair cardiac and lung function in the thorax.
- Ultrafiltration failure: The peritoneal mesothelium is implicated in the long-term development of ultrafiltration failure in peritoneal dialysis patients. The presence of supra-physiological glucose concentrations, acidity, and glucose degradation products in peritoneal dialysis fluids contribute to the fibrosis of the peritoneal mesothelium, either by epithelial–mesenchymal transition or increased proliferation of existing fibroblasts. A fibrosed peritoneum results in the increased passage of solutes across the peritoneum and ultrafiltration failure.
See also[edit]
https://en.wikipedia.org/wiki/Mesothelium
In anatomy, serous membrane (or serosa) is a smooth tissue membrane of mesothelium lining the contents and inner walls of body cavities, which secrete serous fluid to allow lubricated sliding movements between opposing surfaces. The serous membrane that covers internal organs is called a visceral membrane; while the one that covers the cavity wall is called the parietal membrane. Between the two opposing serosal surfaces is often a potential space, mostly empty except for the small amount of serous fluid.[2]
The Latin anatomical name is tunica serosa. Serous membranes line and enclose several body cavities, also known as serous cavities, where they secrete a lubricating fluid which reduces friction from movements. Serosa is entirely different from the adventitia, a connective tissue layer which binds together structures rather than reducing friction between them. The serous membrane covering the heart and lining the mediastinum is referred to as the pericardium, the serous membrane lining the thoracic cavity and surrounding the lungs is referred to as the pleura, and that lining the abdominopelvic cavity and the viscera is referred to as the peritoneum.
https://en.wikipedia.org/wiki/Serous_membrane
The pulmonary pleurae (sing. pleura)[1] are the two opposing layers of serous membrane overlying the lungs and the inside of the surrounding chest walls.
The inner pleura, called the visceral pleura, covers the surface of each lung and dips between the lobes of the lung as fissures, and is formed by the invagination of lung buds into each thoracic sac during embryonic development.[2] The outer layer, called the parietal pleura, lines the inner surfaces of the thoracic cavity on each side of the mediastinum, and can be subdivided into mediastinal (covering the side surfaces of the fibrous pericardium, oesophagus and thoracic aorta), diaphragmatic (covering the upper surface of the diaphragm), costal (covering the inside of rib cage) and cervical (covering the underside of the suprapleural membrane) pleurae. The visceral and the mediastinal parietal pleurae are connected at the root of the lung ("hilum") through a smooth fold known as pleural reflections, and a bell sleeve-like extension of visceral pleura hanging under to the hilum is known as the pulmonary ligament.
Between two pleurae is a potential space called the pleural cavity (also pleural space),[2] which is normally collapsed and filled with only a tiny amount of serous fluid (pleural fluid) secreted by the pleurae, and is clinically considered vacuumousunder healthy conditions. The two lungs bounded by parietal pleura, almost fill the thoracic cavity.
https://en.wikipedia.org/wiki/Pulmonary_pleurae
In the development of the human embryo the intraembryonic coelom (or somatic coelom) is a portion of the conceptusforming in the mesoderm during the third week of development.[1] During the third week of development, the lateral plate mesoderm splits into a dorsal somatic mesoderm (somatopleure) and a ventral splanchnic mesoderm (splanchnopleure). The resulting cavity between the somatopleure and splanchnopleure is called the intraembryonic coelom. This space will give rise to the thoracic and abdominal cavities. The coelomic spaces in the lateral mesoderm and cardiogenic area are isolated. The isolated coelom begins to organize into a horseshoe shape. The spaces soon join together and form a single horseshoe-shaped cavity, the intraembryonic coelom which separates the mesoderm into two layers.[2]
It briefly has a connection with the extraembryonic coelom.
https://en.wikipedia.org/wiki/Intraembryonic_coelom
A joint or articulation (or articular surface) is the connection made between bones, ossicles, or other hard structures in the body which link the skeletal system into a functional whole.[1][2][3] They are constructed to allow for different degrees and types of movement. Some joints, such as the knee, elbow, and shoulder, are self-lubricating, almost frictionless, and are able to withstand compression and maintain heavy loads while still executing smooth and precise movements.[3] Other joints such as sutures between the bones of the skull permit very little movement (only during birth) in order to protect the brain and the sense organs.[3] The connection between a tooth and the jawbone is also called a joint, and is described as a fibrous joint known as a gomphosis. Joints are classified both structurally and functionally.[4]
Joint | |
---|---|
![]() Diagram of a typical synovial joint | |
![]() Depiction of an intervertebral disc, a cartilaginous joint | |
Details | |
System | Musculoskeletal system Articular system |
Identifiers | |
Latin | Articulus Junctura Articulatio |
MeSH | D007596 |
TA98 | A03.0.00.000 |
TA2 | 1515 |
FMA | 7490 |
Anatomical terminology |
Classification[edit]
The number of joints depends on if sesamoids are included, age of the human and the definition of joints. However, the number of sesamoids is the same in most people with variations being rare.[5][6][7]
Joints are mainly classified structurally and functionally. Structural classification is determined by how the bones connect to each other, while functional classification is determined by the degree of movement between the articulating bones. In practice, there is significant overlap between the two types of classifications.
Clinical, numerical classification[edit]
- monoarticular – concerning one joint
- oligoarticular or pauciarticular – concerning 2–4 joints
- polyarticular – concerning 5 or more joints
Structural classification (binding tissue)[edit]
Structural classification names and divides joints according to the type of binding tissue that connects the bones to each other.[1] There are four structural classifications of joints:[8]
- fibrous joint – joined by dense regular connective tissue that is rich in collagen fibers[9]
- cartilaginous joint – joined by cartilage. There are two types: primary cartilaginous joints composed of hyaline cartilage, and secondary cartilaginous joints composed of hyaline cartilage covering the articular surfaces of the involved bones with fibrocartilage connecting them.
- synovial joint – not directly joined – the bones have a synovial cavity and are united by the dense irregular connective tissue that forms the articular capsule that is normally associated with accessory ligaments.[9]
- facet joint – joint between two articular processes between two vertebrae.[10][11]
Functional classification (movement)[edit]
Joints can also be classified functionally according to the type and degree of movement they allow:[1][12] Joint movements are described with reference to the basic anatomical planes.[3]
- synarthrosis – permits little or no mobility. Most synarthrosis joints are fibrous joints (e.g., skull sutures).
- amphiarthrosis – permits slight mobility. Most amphiarthrosis joints are cartilaginous joints (e.g., intervertebral discs).
- synovial joint (also known as a diarthrosis) – freely movable.[1][12] Synovial joints can in turn be classified into six groups according to the type of movement they allow: plane joint, ball and socket joint, hinge joint, pivot joint,[13][14] condyloid joint and saddle joint.[15]
Joints can also be classified, according to the number of axes of movement they allow, into nonaxial (gliding, as between the proximal ends of the ulna and radius), monoaxial (uniaxial), biaxial and multiaxial.[16] Another classification is according to the degrees of freedom allowed, and distinguished between joints with one, two or three degrees of freedom.[16] A further classification is according to the number and shapes of the articular surfaces: flat, concave and convex surfaces.[16] Types of articular surfaces include trochlear surfaces.[17]
Biomechanical classification[edit]
Joints can also be classified based on their anatomy or on their biomechanical properties. According to the anatomic classification, joints are subdivided into simple and compound, depending on the number of bones involved, and into complex and combination joints:[18]
- Simple joint: two articulation surfaces (e.g. shoulder joint, hip joint)
- Compound joint: three or more articulation surfaces (e.g. radiocarpal joint)
- Complex joint: two or more articulation surfaces and an articular disc or meniscus (e.g. knee joint)
Anatomical[edit]
The joints may be classified anatomically into the following groups:
- Joints of hand
- Elbow joints
- Wrist joints
- Axillary joints
- Sternoclavicular joints
- Vertebral articulations
- Temporomandibular joints
- Sacroiliac joints
- Hip joints
- Knee joints
- Articulations of foot
Unmyelinated nerve fibers are abundant in joint capsules and ligaments as well as in the outer part of intraarticular menisci. These nerve fibers are responsible for pain perception when a joint is strained.[19]
Clinical significance[edit]
Damaging the cartilage of joints (articular cartilage) or the bones and muscles that stabilize the joints can lead to joint dislocations and osteoarthritis. Swimming is a great way to exercise the joints with minimal damage.[3]
A joint disorder is termed arthropathy, and when involving inflammation of one or more joints the disorder is called arthritis. Most joint disorders involve arthritis, but joint damage by external physical trauma is typically not termed arthritis.
Arthropathies are called polyarticular (multiarticular) when involving many joints and monoarticular when involving only a single joint.
Arthritis is the leading cause of disability in people over the age of 55. There are many different forms of arthritis, each of which has a different cause. The most common form of arthritis, osteoarthritis (also known as degenerative joint disease), occurs following trauma to the joint, following an infection of the joint or simply as a result of aging and the deterioration of articular cartilage. Furthermore, there is emerging evidence that abnormal anatomy may contribute to early development of osteoarthritis. Other forms of arthritis are rheumatoid arthritis and psoriatic arthritis, which are autoimmune diseases in which the body is attacking itself. Septic arthritisis caused by joint infection. Gouty arthritis is caused by deposition of uric acid crystals in the joint that results in subsequent inflammation. Additionally, there is a less common form of gout that is caused by the formation of rhomboidal-shaped crystals of calcium pyrophosphate. This form of gout is known as pseudogout.
Temporomandibular joint syndrome (TMJ) involves the jaw joints and can cause facial pain, clicking sounds in the jaw, or limitation of jaw movement, to name a few symptoms. It is caused by psychological tension and misalignment of the jaw (malocclusion), and may be affecting as many as 75 million Americans.[3]
History[edit]
Etymology[edit]
The English word joint is a past participle of the verb join, and can be read as joined.[20] Joint is derived from Latin iunctus,[20] past participle of the Latin verb iungere, join, unite, connect, attach.[21]
The English term articulation is derived from Latin articulatio.[20]
Humans have also developed lighter, more fragile joint bones over time due to the decrease in physical activity compared to thousands of years ago.[22]
See also[edit]
https://en.wikipedia.org/wiki/Joint
Cavitation is the formation of cavities, which are spaces or openings in the body. This process occurs in mammalian embryos, and can also occur later on in fully developed organisms. During mammalian embryo development, cavitation is a routine process; however, the formation of cavities in fully developed organs, especially in lung tissue, is usually the sign of a severe medical condition or disease, such as tuberculosis.[1]
Developmental[edit]
Cavitation is a crucial process in the development of mammalian embryos.[2] After fertilization, rapid cell division occurs which results in the formation of the morula, or a solid ball of cells. The morula is the precursor structure to the blastula, which is an animal embryo in the early stages of development. The morula consists of a cluster of internal cells covered by a layer of external cells. The internal cells become the inner cellular mass, which becomes the entire embryo. The external cells are destined to become a structure called the trophoblast, a layer of tissue on the inside of the embryo that provides it with nourishment. The trophoblast cells become extraembryonic structures necessary for development. After the initial formation of the morula, it does not have an interior space or cavity. Cavitation occurs to create a cavity on the inside of the morula. This process occurs when trophoblast cells, in other words the outside covering of the blastocyst, secretes fluid into the morula creating the blastocoel, the fluid filled cavity of the blastula.[3] The formation of the blastocoel is a critical stage in the formation of the blastocyst,[4] which is a blastula where some cellular differentiation has already occurred.[5][6]
https://en.wikipedia.org/wiki/Cavitation_(embryology)
Cellular differentiation is the process in which a stem cell alters from one type to a differentiated one[2][3] Usually, the cell changes to a more specialized type. Differentiation happens multiple times during the development of a multicellular organism as it changes from a simple zygote to a complex system of tissues and cell types. Differentiation continues in adulthood as adult stem cells divide and create fully differentiated daughter cells during tissue repair and during normal cell turnover. Some differentiation occurs in response to antigen exposure. Differentiation dramatically changes a cell's size, shape, membrane potential, metabolic activity, and responsiveness to signals. These changes are largely due to highly controlled modifications in gene expressionand are the study of epigenetics. With a few exceptions, cellular differentiation almost never involves a change in the DNA sequence itself. Although metabolic composition does get altered quite dramatically[4] where stem cells are characterized by abundant metabolites with highly unsaturated structures whose levels decrease upon differentiation. Thus, different cells can have very different physical characteristics despite having the same genome.
A specialized type of differentiation, known as terminal differentiation, is of importance in some tissues, for example vertebrate nervous system, striated muscle, epidermis and gut. During terminal differentiation, a precursor cell formerly capable of cell division, permanently leaves the cell cycle, dismantles the cell cycle machinery and often expresses a range of genes characteristic of the cell's final function (e.g. myosin and actin for a muscle cell). Differentiation may continue to occur after terminal differentiation if the capacity and functions of the cell undergo further changes.
Among dividing cells, there are multiple levels of cell potency, the cell's ability to differentiate into other cell types. A greater potency indicates a larger number of cell types that can be derived. A cell that can differentiate into all cell types, including the placental tissue, is known as totipotent. In mammals, only the zygote and subsequent blastomeres are totipotent, while in plants, many differentiated cells can become totipotent with simple laboratory techniques. A cell that can differentiate into all cell types of the adult organism is known as pluripotent. Such cells are called meristematic cells in higher plants and embryonic stem cells in animals, though some groups report the presence of adult pluripotent cells. Virally induced expression of four transcription factors Oct4, Sox2, c-Myc, and Klf4 (Yamanaka factors) is sufficient to create pluripotent (iPS) cells from adult fibroblasts.[5] A multipotent cell is one that can differentiate into multiple different, but closely related cell types.[6] Oligopotent cells are more restricted than multipotent, but can still differentiate into a few closely related cell types.[6] Finally, unipotent cells can differentiate into only one cell type, but are capable of self-renewal.[6] In cytopathology, the level of cellular differentiation is used as a measure of cancer progression. "Grade" is a marker of how differentiated a cell in a tumor is.[7]
Mammalian cell types[edit]
Three basic categories of cells make up the mammalian body: germ cells, somatic cells, and stem cells. Each of the approximately 37.2 trillion (3.72x1013) cells in an adult human has its own copy or copies of the genome except certain cell types, such as red blood cells, that lack nuclei in their fully differentiated state. Most cells are diploid; they have two copies of each chromosome. Such cells, called somatic cells, make up most of the human body, such as skin and muscle cells. Cells differentiate to specialize for different functions.[8]
Germ line cells are any line of cells that give rise to gametes—eggs and sperm—and thus are continuous through the generations. Stem cells, on the other hand, have the ability to divide for indefinite periods and to give rise to specialized cells. They are best described in the context of normal human development.[citation needed]
Development begins when a sperm fertilizes an egg and creates a single cell that has the potential to form an entire organism. In the first hours after fertilization, this cell divides into identical cells. In humans, approximately four days after fertilization and after several cycles of cell division, these cells begin to specialize, forming a hollow sphere of cells, called a blastocyst.[9] The blastocyst has an outer layer of cells, and inside this hollow sphere, there is a cluster of cells called the inner cell mass. The cells of the inner cell mass go on to form virtually all of the tissues of the human body. Although the cells of the inner cell mass can form virtually every type of cell found in the human body, they cannot form an organism. These cells are referred to as pluripotent.[10]
Pluripotent stem cells undergo further specialization into multipotent progenitor cells that then give rise to functional cells. Examples of stem and progenitor cells include:[citation needed]
- Radial glial cells (embryonic neural stem cells) that give rise to excitatory neurons in the fetal brain through the process of neurogenesis.[11][12][13]
- Hematopoietic stem cells (adult stem cells) from the bone marrow that give rise to red blood cells, white blood cells, and platelets
- Mesenchymal stem cells (adult stem cells) from the bone marrow that give rise to stromal cells, fat cells, and types of bone cells
- Epithelial stem cells (progenitor cells) that give rise to the various types of skin cells
- Muscle satellite cells (progenitor cells) that contribute to differentiated muscle tissue.
A pathway that is guided by the cell adhesion molecules consisting of four amino acids, arginine, glycine, asparagine, and serine, is created as the cellular blastomere differentiates from the single-layered blastula to the three primary layers of germ cells in mammals, namely the ectoderm, mesoderm and endoderm (listed from most distal (exterior) to proximal (interior)). The ectoderm ends up forming the skin and the nervous system, the mesoderm forms the bones and muscular tissue, and the endoderm forms the internal organ tissues.
Dedifferentiation[edit]
Dedifferentiation, or integration, is a cellular process often seen in more basal life forms such as worms and amphibians in which a partially or terminally differentiated cell reverts to an earlier developmental stage, usually as part of a regenerativeprocess.[14][15] Dedifferentiation also occurs in plants.[16] Cells in cell culture can lose properties they originally had, such as protein expression, or change shape. This process is also termed dedifferentiation.[17]
Some believe dedifferentiation is an aberration of the normal development cycle that results in cancer,[18] whereas others believe it to be a natural part of the immune response lost by humans at some point as a result of evolution.
A small molecule dubbed reversine, a purine analog, has been discovered that has proven to induce dedifferentiation in myotubes. These dedifferentiated cells could then redifferentiate into osteoblasts and adipocytes.[19]
Mechanisms[edit]
Each specialized cell type in an organism expresses a subset of all the genes that constitute the genome of that species. Each cell type is defined by its particular pattern of regulated gene expression. Cell differentiation is thus a transition of a cell from one cell type to another and it involves a switch from one pattern of gene expression to another. Cellular differentiation during development can be understood as the result of a gene regulatory network. A regulatory gene and its cis-regulatory modules are nodes in a gene regulatory network; they receive input and create output elsewhere in the network.[20] The systems biology approach to developmental biology emphasizes the importance of investigating how developmental mechanisms interact to produce predictable patterns (morphogenesis). However, an alternative view has been proposed recently[when?][by whom?]. Based on stochastic gene expression, cellular differentiation is the result of a Darwinian selective process occurring among cells. In this frame, protein and gene networks are the result of cellular processes and not their cause.[citation needed]
While evolutionarily conserved molecular processes are involved in the cellular mechanisms underlying these switches, in animal species these are very different from the well-characterized gene regulatory mechanisms of bacteria, and even from those of the animals' closest unicellular relatives.[21] Specifically, cell differentiation in animals is highly dependent on biomolecular condensates of regulatory proteins and enhancer DNA sequences.
Cellular differentiation is often controlled by cell signaling. Many of the signal molecules that convey information from cell to cell during the control of cellular differentiation are called growth factors. Although the details of specific signal transduction pathways vary, these pathways often share the following general steps. A ligand produced by one cell binds to a receptor in the extracellular region of another cell, inducing a conformational change in the receptor. The shape of the cytoplasmic domain of the receptor changes, and the receptor acquires enzymatic activity. The receptor then catalyzes reactions that phosphorylate other proteins, activating them. A cascade of phosphorylation reactions eventually activates a dormant transcription factor or cytoskeletal protein, thus contributing to the differentiation process in the target cell.[22] Cells and tissues can vary in competence, their ability to respond to external signals.[23]
Signal induction refers to cascades of signaling events, during which a cell or tissue signals to another cell or tissue to influence its developmental fate.[23] Yamamoto and Jeffery[24] investigated the role of the lens in eye formation in cave- and surface-dwelling fish, a striking example of induction.[23] Through reciprocal transplants, Yamamoto and Jeffery[24] found that the lens vesicle of surface fish can induce other parts of the eye to develop in cave- and surface-dwelling fish, while the lens vesicle of the cave-dwelling fish cannot.[23]
Other important mechanisms fall under the category of asymmetric cell divisions, divisions that give rise to daughter cells with distinct developmental fates. Asymmetric cell divisions can occur because of asymmetrically expressed maternal cytoplasmic determinants or because of signaling.[23] In the former mechanism, distinct daughter cells are created during cytokinesis because of an uneven distribution of regulatory molecules in the parent cell; the distinct cytoplasm that each daughter cell inherits results in a distinct pattern of differentiation for each daughter cell. A well-studied example of pattern formation by asymmetric divisions is body axis patterning in Drosophila. RNA molecules are an important type of intracellular differentiation control signal. The molecular and genetic basis of asymmetric cell divisions has also been studied in green algae of the genus Volvox, a model system for studying how unicellular organisms can evolve into multicellular organisms.[23] In Volvox carteri, the 16 cells in the anterior hemisphere of a 32-cell embryo divide asymmetrically, each producing one large and one small daughter cell. The size of the cell at the end of all cell divisions determines whether it becomes a specialized germ or somatic cell.[23][25]
Epigenetic control[edit]
Since each cell, regardless of cell type, possesses the same genome, determination of cell type must occur at the level of gene expression. While the regulation of gene expression can occur through cis- and trans-regulatory elements including a gene's promoter and enhancers, the problem arises as to how this expression pattern is maintained over numerous generations of cell division. As it turns out, epigenetic processes play a crucial role in regulating the decision to adopt a stem, progenitor, or mature cell fate. This section will focus primarily on mammalian stem cells.
In systems biology and mathematical modeling of gene regulatory networks, cell-fate determination is predicted to exhibit certain dynamics, such as attractor-convergence (the attractor can be an equilibrium point, limit cycle or strange attractor) or oscillatory.[26]
Importance of epigenetic control[edit]
The first question that can be asked is the extent and complexity of the role of epigenetic processes in the determination of cell fate. A clear answer to this question can be seen in the 2011 paper by Lister R, et al. [27] on aberrant epigenomic programming in human induced pluripotent stem cells. As induced pluripotent stem cells (iPSCs) are thought to mimic embryonic stem cells in their pluripotent properties, few epigenetic differences should exist between them. To test this prediction, the authors conducted whole-genome profiling of DNA methylation patterns in several human embryonic stem cell (ESC), iPSC, and progenitor cell lines.
Female adipose cells, lung fibroblasts, and foreskin fibroblasts were reprogrammed into induced pluripotent state with the OCT4, SOX2, KLF4, and MYC genes. Patterns of DNA methylation in ESCs, iPSCs, somatic cells were compared. Lister R, et al. observed significant resemblance in methylation levels between embryonic and induced pluripotent cells. Around 80% of CG dinucleotides in ESCs and iPSCs were methylated, the same was true of only 60% of CG dinucleotides in somatic cells. In addition, somatic cells possessed minimal levels of cytosine methylation in non-CG dinucleotides, while induced pluripotent cells possessed similar levels of methylation as embryonic stem cells, between 0.5 and 1.5%. Thus, consistent with their respective transcriptional activities,[27] DNA methylation patterns, at least on the genomic level, are similar between ESCs and iPSCs.
However, upon examining methylation patterns more closely, the authors discovered 1175 regions of differential CG dinucleotide methylation between at least one ES or iPS cell line. By comparing these regions of differential methylation with regions of cytosine methylation in the original somatic cells, 44-49% of differentially methylated regions reflected methylation patterns of the respective progenitor somatic cells, while 51-56% of these regions were dissimilar to both the progenitor and embryonic cell lines. In vitro-induced differentiation of iPSC lines saw transmission of 88% and 46% of hyper and hypo-methylated differentially methylated regions, respectively.
Two conclusions are readily apparent from this study. First, epigenetic processes are heavily involved in cell fate determination, as seen from the similar levels of cytosine methylation between induced pluripotent and embryonic stem cells, consistent with their respective patterns of transcription. Second, the mechanisms of reprogramming (and by extension, differentiation) are very complex and cannot be easily duplicated, as seen by the significant number of differentially methylated regions between ES and iPS cell lines. Now that these two points have been established, we can examine some of the epigenetic mechanisms that are thought to regulate cellular differentiation.
Mechanisms of epigenetic regulation[edit]
Pioneer factors (Oct4, Sox2, Nanog)[edit]
Three transcription factors, OCT4, SOX2, and NANOG – the first two of which are used in induced pluripotent stem cell (iPSC) reprogramming, along with Klf4 and c-Myc – are highly expressed in undifferentiated embryonic stem cells and are necessary for the maintenance of their pluripotency.[28] It is thought that they achieve this through alterations in chromatin structure, such as histone modification and DNA methylation, to restrict or permit the transcription of target genes. While highly expressed, their levels require a precise balance to maintain pluripotency, perturbation of which will promote differentiation towards different lineages based on how the gene expression levels change. Differential regulation of Oct-4 and SOX2 levels have been shown to precede germ layer fate selection.[29] Increased levels of Oct4 and decreased levels of Sox2 promote a mesendodermal fate, with Oct4 actively suppressing genes associated with a neural ectodermal fate. Similarly, Increased levels of Sox2 and decreased levels of Oct4 promote differentiation towards a neural ectodermal fate, with Sox2 inhibiting differentiation towards a mesendodermal fate. Regardless of the lineage cells differentiate down, suppression of NANOG has been identified as a necessary prerequisite for differentiation.[29]
Polycomb repressive complex (PRC2)[edit]
In the realm of gene silencing, Polycomb repressive complex 2, one of two classes of the Polycomb group (PcG) family of proteins, catalyzes the di- and tri-methylation of histone H3 lysine 27 (H3K27me2/me3).[28][30][31] By binding to the H3K27me2/3-tagged nucleosome, PRC1 (also a complex of PcG family proteins) catalyzes the mono-ubiquitinylation of histone H2A at lysine 119 (H2AK119Ub1), blocking RNA polymerase II activity and resulting in transcriptional suppression.[28] PcG knockout ES cells do not differentiate efficiently into the three germ layers, and deletion of the PRC1 and PRC2 genes leads to increased expression of lineage-affiliated genes and unscheduled differentiation.[28] Presumably, PcG complexes are responsible for transcriptionally repressing differentiation and development-promoting genes.
Trithorax group proteins (TrxG)[edit]
Alternately, upon receiving differentiation signals, PcG proteins are recruited to promoters of pluripotency transcription factors. PcG-deficient ES cells can begin differentiation but cannot maintain the differentiated phenotype.[28] Simultaneously, differentiation and development-promoting genes are activated by Trithorax group (TrxG) chromatin regulators and lose their repression.[28][31] TrxG proteins are recruited at regions of high transcriptional activity, where they catalyze the trimethylation of histone H3 lysine 4 (H3K4me3) and promote gene activation through histone acetylation.[31] PcG and TrxG complexes engage in direct competition and are thought to be functionally antagonistic, creating at differentiation and development-promoting loci what is termed a "bivalent domain" and rendering these genes sensitive to rapid induction or repression.[32]
DNA methylation[edit]
Regulation of gene expression is further achieved through DNA methylation, in which the DNA methyltransferase-mediated methylation of cytosine residues in CpG dinucleotides maintains heritable repression by controlling DNA accessibility.[32] The majority of CpG sites in embryonic stem cells are unmethylated and appear to be associated with H3K4me3-carrying nucleosomes.[28] Upon differentiation, a small number of genes, including OCT4 and NANOG,[32] are methylated and their promoters repressed to prevent their further expression. Consistently, DNA methylation-deficient embryonic stem cells rapidly enter apoptosis upon in vitro differentiation.[28]
Nucleosome positioning[edit]
While the DNA sequence of most cells of an organism is the same, the binding patterns of transcription factors and the corresponding gene expression patterns are different. To a large extent, differences in transcription factor binding are determined by the chromatin accessibility of their binding sites through histone modificationand/or pioneer factors. In particular, it is important to know whether a nucleosome is covering a given genomic binding site or not. This can be determined using a chromatin immunoprecipitation (ChIP) assay.[33]
Histone acetylation and methylation[edit]
DNA-nucleosome interactions are characterized by two states: either tightly bound by nucleosomes and transcriptionally inactive, called heterochromatin, or loosely bound and usually, but not always, transcriptionally active, called euchromatin. The epigenetic processes of histone methylation and acetylation, and their inverses demethylation and deacetylation primarily account for these changes. The effects of acetylation and deacetylation are more predictable. An acetyl group is either added to or removed from the positively charged Lysine residues in histones by enzymes called histone acetyltransferases or histone deacteylases, respectively. The acetyl group prevents Lysine's association with the negatively charged DNA backbone. Methylation is not as straightforward, as neither methylation nor demethylation consistently correlate with either gene activation or repression. However, certain methylations have been repeatedly shown to either activate or repress genes. The trimethylation of lysine 4 on histone 3 (H3K4Me3) is associated with gene activation, whereas trimethylation of lysine 27 on histone 3 represses genes[34][35][36]
In stem cells[edit]
During differentiation, stem cells change their gene expression profiles. Recent studies have implicated a role for nucleosome positioning and histone modifications during this process.[37] There are two components of this process: turning off the expression of embryonic stem cell (ESC) genes, and the activation of cell fate genes. Lysine specific demethylase 1 (KDM1A) is thought to prevent the use of enhancer regions of pluripotency genes, thereby inhibiting their transcription.[38] It interacts with Mi-2/NuRD complex (nucleosome remodelling and histone deacetylase) complex,[38] giving an instance where methylation and acetylation are not discrete and mutually exclusive, but intertwined processes.
Role of signaling in epigenetic control[edit]
A final question to ask concerns the role of cell signaling in influencing the epigenetic processes governing differentiation. Such a role should exist, as it would be reasonable to think that extrinsic signaling can lead to epigenetic remodeling, just as it can lead to changes in gene expression through the activation or repression of different transcription factors. Little direct data is available concerning the specific signals that influence the epigenome, and the majority of current knowledge about the subject consists of speculations on plausible candidate regulators of epigenetic remodeling.[39] We will first discuss several major candidates thought to be involved in the induction and maintenance of both embryonic stem cells and their differentiated progeny, and then turn to one example of specific signaling pathways in which more direct evidence exists for its role in epigenetic change.
The first major candidate is Wnt signaling pathway. The Wnt pathway is involved in all stages of differentiation, and the ligand Wnt3a can substitute for the overexpression of c-Myc in the generation of induced pluripotent stem cells.[39] On the other hand, disruption of β-catenin, a component of the Wnt signaling pathway, leads to decreased proliferation of neural progenitors.
Growth factors comprise the second major set of candidates of epigenetic regulators of cellular differentiation. These morphogens are crucial for development, and include bone morphogenetic proteins, transforming growth factors (TGFs), and fibroblast growth factors (FGFs). TGFs and FGFs have been shown to sustain expression of OCT4, SOX2, and NANOG by downstream signaling to Smad proteins.[39] Depletion of growth factors promotes the differentiation of ESCs, while genes with bivalent chromatin can become either more restrictive or permissive in their transcription.[39]
Several other signaling pathways are also considered to be primary candidates. Cytokine leukemia inhibitory factors are associated with the maintenance of mouse ESCs in an undifferentiated state. This is achieved through its activation of the Jak-STAT3 pathway, which has been shown to be necessary and sufficient towards maintaining mouse ESC pluripotency.[40] Retinoic acid can induce differentiation of human and mouse ESCs,[39] and Notch signaling is involved in the proliferation and self-renewal of stem cells. Finally, Sonic hedgehog, in addition to its role as a morphogen, promotes embryonic stem cell differentiation and the self-renewal of somatic stem cells.[39]
The problem, of course, is that the candidacy of these signaling pathways was inferred primarily on the basis of their role in development and cellular differentiation. While epigenetic regulation is necessary for driving cellular differentiation, they are certainly not sufficient for this process. Direct modulation of gene expression through modification of transcription factors plays a key role that must be distinguished from heritable epigenetic changes that can persist even in the absence of the original environmental signals. Only a few examples of signaling pathways leading to epigenetic changes that alter cell fate currently exist, and we will focus on one of them.
Expression of Shh (Sonic hedgehog) upregulates the production of BMI1, a component of the PcG complex that recognizes H3K27me3. This occurs in a Gli-dependent manner, as Gli1 and Gli2 are downstream effectors of the Hedgehog signaling pathway. In culture, Bmi1 mediates the Hedgehog pathway's ability to promote human mammary stem cell self-renewal.[41] In both humans and mice, researchers showed Bmi1 to be highly expressed in proliferating immature cerebellar granule cell precursors. When Bmi1 was knocked out in mice, impaired cerebellar development resulted, leading to significant reductions in postnatal brain mass along with abnormalities in motor control and behavior.[42] A separate study showed a significant decrease in neural stem cell proliferation along with increased astrocyte proliferation in Bmi null mice.[43]
An alternative model of cellular differentiation during embryogenesis is that positional information is based on mechanical signalling by the cytoskeleton using Embryonic differentiation waves. The mechanical signal is then epigenetically transduced via signal transduction systems (of which specific molecules such as Wnt are part) to result in differential gene expression.
In summary, the role of signaling in the epigenetic control of cell fate in mammals is largely unknown, but distinct examples exist that indicate the likely existence of further such mechanisms.
Effect of matrix elasticity[edit]
In order to fulfill the purpose of regenerating a variety of tissues, adult stems are known to migrate from their niches, adhere to new extracellular matrices (ECM) and differentiate. The ductility of these microenvironments are unique to different tissue types. The ECM surrounding brain, muscle and bone tissues range from soft to stiff. The transduction of the stem cells into these cells types is not directed solely by chemokine cues and cell to cell signaling. The elasticity of the microenvironment can also affect the differentiation of mesenchymal stem cells (MSCs which originate in bone marrow.) When MSCs are placed on substrates of the same stiffness as brain, muscle and bone ECM, the MSCs take on properties of those respective cell types.[44] Matrix sensing requires the cell to pull against the matrix at focal adhesions, which triggers a cellular mechano-transducer to generate a signal to be informed what force is needed to deform the matrix. To determine the key players in matrix-elasticity-driven lineage specification in MSCs, different matrix microenvironments were mimicked. From these experiments, it was concluded that focal adhesions of the MSCs were the cellular mechano-transducer sensing the differences of the matrix elasticity. The non-muscle myosin IIa-c isoforms generates the forces in the cell that lead to signaling of early commitment markers. Nonmuscle myosin IIa generates the least force increasing to non-muscle myosin IIc. There are also factors in the cell that inhibit non-muscle myosin II, such as blebbistatin. This makes the cell effectively blind to the surrounding matrix.[44] Researchers have obtained some success in inducing stem cell-like properties in HEK 239 cells by providing a soft matrix without the use of diffusing factors.[45] The stem-cell properties appear to be linked to tension in the cells' actin network. One identified mechanism for matrix-induced differentiation is tension-induced proteins, which remodel chromatin in response to mechanical stretch.[46] The RhoA pathway is also implicated in this process.
Evolutionary history[edit]
A billion-years-old, likely holozoan, protist, Bicellum brasieri with two types of cells, shows that the evolution of differentiated multicellularity, possibly but not necessarily of animal lineages, occurred at least 1 billion years ago and possibly mainly in freshwater lakes rather than the ocean.[47][48][49][clarification needed]
See also[edit]
- Interbilayer Forces in Membrane Fusion
- Fusion mechanism
- Lipid bilayer fusion
- Cell-cell fusogens
- CAF-1
- List of human cell types derived from the germ layers
https://en.wikipedia.org/wiki/Cellular_differentiation
Cell–cell fusogens are glycoproteins that facilitate the fusion of cell to cell membranes. Cell–cell fusion is critical for the merging of gamete genomes and the development of organs in multicellular organisms. Cell-cell fusion occurs when both actin cytoskeleton and fusogenic proteins properly rearrange across the cell membrane. This process is led by actin-propelled membrane protrusions.[1]
https://en.wikipedia.org/wiki/Cell–cell_fusogens
In membrane biology, fusion is the process by which two initially distinct lipid bilayers merge their hydrophobiccores, resulting in one interconnected structure. If this fusion proceeds completely through both leaflets of both bilayers, an aqueous bridge is formed and the internal contents of the two structures can mix. Alternatively, if only one leaflet from each bilayer is involved in the fusion process, the bilayers are said to be hemifused. In hemifusion, the lipid constituents of the outer leaflet of the two bilayers can mix, but the inner leaflets remain distinct. The aqueous contents enclosed by each bilayer also remain separated.
Fusion is involved in many cellular processes, particularly in eukaryotes since the eukaryotic cell is extensively sub-divided by lipid bilayer membranes. Exocytosis, fertilization of an egg by sperm and transport of waste products to the lysosome are a few of the many eukaryotic processes that rely on some form of fusion. Fusion is also an important mechanism for transport of lipids from their site of synthesis to the membrane where they are needed. Even the entry of pathogens can be governed by fusion, as many bilayer-coated viruses have dedicated fusion proteins to gain entry into the host cell.
https://en.wikipedia.org/wiki/Lipid_bilayer_fusion
A fusion mechanism is any mechanism by which cell fusion or virus–cell fusion takes place, as well as the machinery that facilitates these processes. Cell fusion is the formation of a hybrid cell from two separate cells.[1][2] There are three major actions taken in both virus–cell fusion and cell–cell fusion: the dehydration of polar head groups, the promotion of a hemifusion stalk, and the opening and expansion of pores between fusing cells.[3] Virus–cell fusions occur during infections of several viruses that are health concerns relevant today. Some of these include HIV, Ebola, and influenza.[4] For example, HIV infects by fusing with the membranes of immune system cells. In order for HIV to fuse with a cell, it must be able to bind to the receptors CD4, CCR5, and CXCR4. Cell fusion also occurs in a multitude of mammalian cells including gametes and myoblasts.[5]
https://en.wikipedia.org/wiki/Fusion_mechanism
Class I fusogens[edit]
These fusogens are trimeric, meaning they are made of three subunits. Their fusion loops are hidden internally at the junctions of the monomers before fusion takes place. Once fusion is complete, they refold into a different trimeric structure than the structure they had before fusion. These fusogens are characterized by a group of six α-helices in their post-fusion structure. This class of fusogens contains some of the proteins utilized by influenza, HIV, coronaviruses, and Ebola during infection. This class of fusogens also includes syncytins, which are utilized in mammalian cell fusions.[8][4][9]
Class II fusogens[edit]
Class II fusogens contain multiple β-pleated sheets. These proteins are also trimeric and take part in the insertion of fusion loops into the target membrane. Their conformation changes can be triggered by exposure to acidic environments.[8][4] Class II fusogens have a structure distinct from Class I fusogens, but similarly lower the energy barrier for membrane fusion. Class I fusogens are involved in flaviviruses (tick-borne encephalitis); alphaviruses (Semliki Forest virus, Sindbis virus, chikungunya and rubella); and phleboviruses (Rift Valley fever virus and Uukuniemi virus).[8]
Class III fusogens[edit]
Class III fusogens are involved with virus–cell fusions. Much like fusogens in the previous two classes, these proteins are trimeric. However, they contain both α-helices and β-pleated sheets. During cell fusion the monomers of these proteins will dissociate but will return to a different trimeric structure after the fusion is complete. They are also involved in the insertion of fusion loops in the membrane.[4]
Class IV fusogens[edit]
These reoviral cell–cell fusogens contain fusion loops that can induce cell fusion. They form polymeric structures to induce fusion of membranes. Reoviruses do not have membranes themselves, so class IV fusogens are not usually involved in traditional virus–cell fusion. However, when they are expressed on the surface of cells, they can induce cell–cell fusion.[4]
Class I–III mechanism[edit]
The fusogens of classes I–III have many structural differences. However, the method they utilize to induce membrane fusion is mechanistically similar. When activated, all of these fusogens form elongated trimeric structures and bury their fusion peptides into the membrane of the target cell. They are secured in the viral membrane by hydrophobic trans-membrane regions. These fusogens will then fold in on themselves forming a structure that is reminiscent of a hairpin.[4] This folding action brings the transmembrane region and the fusion loop adjacent to each other. Consequently, the viral membrane and the target cell membrane are also pulled close together.[6] As the membranes are brought closer together, they are dehydrated, which allows the membranes to be brought into contact.[3] Interactions between hydrophobic amino-acid residues and the adjacent membranes cause destabilization of the membranes. This allows the phospholipids in the outer layer of each membrane to interact with each other. The outer leaflets of the two membranes form a hemifusion stalk to minimize energetically unfavorable interactions between hydrophobic phospholipid tails and the environment. This stalk expands, allowing the inner leaflets of each membrane to interact. These inner leaflets then fuse, forming a fusion pore. At this point, the cytoplasmic components of the cell and the virus begin to mix. As the fusion pore expands, virus–cell fusion is completed.[6]
Mammalian cell fusion mechanisms[edit]
Though there is much variation in different fusions between mammalian cells, there are five stages that occur in a majority of these fusion events: "programming fusion-competent status, chemotaxis, membrane adhesion, membrane fusion, and post-fusion resetting."[5]
Programming fusion-competent status[edit]
This first step, also known as priming, encompasses the necessary events that must take place in order for cells to gain the ability to fuse. In order for a cell to become fusion-competent, it must manipulate the make-up of its membrane to facilitate membrane fusion. It also must construct necessary proteins to mediate fusion. Finally, it must eliminate hindrances to fusion. For example, a cell might free itself from the extracellular matrix in order to allow the cell more motility to facilitate fusion.[5]
Monocytes, macrophages, and osteoclasts[edit]
Monocytes and macrophages can become fusion-competent in response to cytokines, which are protein-signalling molecules. Some interleukins prompt monocytes and macrophages to fuse to form foreign-body giant cells as part of a body's immune response. For example, interleukin-4 can promote the activation of transcription factor STAT6 by phosphorylation. This can then trigger expression of matrix metalloproteinase 9 (MMP9).[5] MMP9 can degrade proteins in the extracellular matrix, which aids in the priming of macrophages for fusion.[7]
Osteoclasts are multinucleated bone-resorbing cells. They are formed by the fusion of differentiated monocytes, much like foreign-body giant cells. However, the molecules that induce fusion-competence in macrophages that are destined to become osteoclasts are different from those that promote formation of foreign-body giant cells. For instance, transcription factor NFATC1 regulates genes that are specific to osteoclast differentiation.[5]
Haploid cells[edit]
Zygote formation is a crucial step in sexual reproduction, and it is reliant on the fusion of sperm and egg cells. Consequently, these cells must be primed to gain fusion-competence. Phosphatidylserine is a phospholipid that usually resides on the inner layer of the cell membrane. After sperm cells are primed, phosphatidylserine can be found on the outer leaflet of the membrane. It is thought that this helps stabilize the membrane at the head of the sperm, and that it may play a role in allowing the sperm to enter the zona pellucida that covers egg cells. This unusual location of phosphatidylserine is an example of membrane restructuring during priming for cell fusion.[5]
Chemotaxis[edit]
Chemotaxis is the process of recruitment in response to the presence of certain signal molecules. Cells that are destined to fuse are attracted to each other via chemotaxis. For example, sperm cells are attracted to the egg cell through signalling by progesterone.[5] Similarly, in muscle tissue, myoblasts can be recruited for fusion by IL-4.[7]
Membrane adhesion[edit]
Before cells can fuse, they must be in contact with one another. This can be accomplished through cell recognition and attachment by cellular machinery.[5] Syncytin-1is a Class I fusogen involved in the fusion of cells to form osteoclasts in humans.[10] During the early actions of Class I fusogens in cell fusion, they insert their fusion loops into a target membrane. Consequently, the action of syncytin-1 is an example of membrane adhesion as it links the two cells together to prepare them for fusion.[6] This step also encompasses the dehydration of the membranes at the site of fusion. This is necessary to overcome the energy requirements necessary for fusion and to ensure that the membranes are in very close proximity for fusion to occur.[3]
Membrane fusion[edit]
Membrane fusion is characterized by the formation of a fusion pore, which allows the internal contents of both cells to mix.[5] It is first accomplished by the mixing of lipids of the outer layers of the fusing membranes, which forms a hemifusion stalk.[6] Then the inner leaflets can interact and fuse, creating an open gap where the membranes have fused. This gap is the fusion pore. This process is mediated by fusogens.[5] Fusogens are highly conserved in mammals, and it is theorized that mammals adopted them after infection by retroviruses.[7] Because they are highly conserved, they perform their task through a similar mechanism to the one used by viral fusogens as previously described.[6] It is theorized that actin polymerization and other actions of the cytoskeleton might aid in the widening of the fusion pore to complete fusion.[5]
Post-fusion resetting[edit]
Upon the completion of fusion, the machinery used to fuse must be disassembled or altered to avoid fusion of the new, multinucleated cell with more cells.[5] One example of this is the final trimeric structure taken on by Class I, II, and III fusogens. They each take on a structure that is markedly different than their form before fusion occurred.[4] This likely alters their activity, preventing them from initiating another fusion.[citation needed]
See also[edit]
Multinucleate cells (multinucleated or polynuclear cells) are eukaryotic cells that have more than one nucleus per cell, i.e., multiple nuclei share one common cytoplasm. Mitosis in multinucleate cells can occur either in a coordinated, synchronous manner where all nuclei divide simultaneously or asynchronously where individual nuclei divide independently in time and space. Certain organisms may have a multinuclear stage of their life cycle. For example, slime molds have a vegetative, multinucleate life stage called a plasmodium.[1]
Although not normally viewed as a case of multinucleation, plant cells share a common cytoplasm by plasmodesmata, and most cells in animal tissues are in communication with their neighbors via gap junctions.[2]
Multinucleate cells, depending on the mechanism by which they are formed, can be divided into[3][4] "syncytia" (formed by cell fusion) or "coenocytes" (formed by nuclear division not being followed by cytokinesis).
A number of dinoflagellates are known to have two nuclei. Unlike other multinucleated cells these nuclei contain two distinct lineages of DNA: one from the dinoflagellate and the other from a symbiotic diatom.[5]
Some bacteria, such as Mycoplasma pneumoniae, a pathogen of the respiratory tract, may display multinuclear filaments as a result of a delay between genome replication and cellular division.[6]
https://en.wikipedia.org/wiki/Multinucleate
Chromatin assembly factor-1 (CAF-1) is a protein complex — including Chaf1a (p150), Chaf1b (p60), and p48 subunits in humans, or Cac1, Cac2, and Cac3, respectively, in yeast— that assembles histone tetramers onto replicating DNA during the S phase of the cell cycle.[1][2][3][4]
https://en.wikipedia.org/wiki/Chromatin_assembly_factor_1
A nucleosome is the basic structural unit of DNA packaging in eukaryotes. The structure of a nucleosome consists of a segment of DNA wound around eight histone proteins[1] and resembles thread wrapped around a spool. The nucleosome is the fundamental subunit of chromatin. Each nucleosome is composed of a little less than two turns of DNA wrapped around a set of eight proteins called histones, which are known as a histone octamer. Each histone octamer is composed of two copies each of the histone proteins H2A, H2B, H3, and H4.
https://en.wikipedia.org/wiki/Nucleosome
The dinoflagellates (Greek δῖνος dinos "whirling" and Latin flagellum "whip, scourge") are a monophyletic group of single-celled eukaryotes constituting the phylum Dinoflagellata[5] and are usually considered algae. Dinoflagellates are mostly marineplankton, but they also are common in freshwater habitats. Their populations vary with sea surface temperature, salinity, and depth. Many dinoflagellates are photosynthetic, but a large fraction of these are in fact mixotrophic, combining photosynthesis with ingestion of prey (phagotrophy and myzocytosis).[6][7]
In terms of number of species, dinoflagellates are one of the largest groups of marine eukaryotes, although substantially smaller than diatoms.[8] Some species are endosymbionts of marine animals and play an important part in the biology of coral reefs. Other dinoflagellates are unpigmented predators on other protozoa, and a few forms are parasitic (for example, Oodinium and Pfiesteria). Some dinoflagellates produce resting stages, called dinoflagellate cysts or dinocysts, as part of their lifecycles, and are known from 84 of the 350 described freshwater species, and from a little more than 10% of the known marine species.[9][10] Dinoflagellates are alveolates possessing two flagella, the ancestral condition of bikonts.
About 1,555 species of free-living marine dinoflagellates are currently described.[11] Another estimate suggests about 2,000 living species, of which more than 1,700 are marine (free-living, as well as benthic) and about 220 are from fresh water.[12] The latest estimates suggest a total of 2,294 living dinoflagellate species, which includes marine, freshwater, and parasitic dinoflagellates.[2]
A rapid accumulation of certain dinoflagellates can result in a visible coloration of the water, colloquially known as red tide (a harmful algal bloom), which can cause shellfish poisoning if humans eat contaminated shellfish. Some dinoflagellates also exhibit bioluminescence—primarily emitting blue-green light. Thus, some parts of the ocean light up at night giving blue-green light.
https://en.wikipedia.org/wiki/Dinoflagellate
Diatom (Neo-Latin diatoma)[a] refers to any member of a large group comprising several genera of algae, specifically microalgae, found in the oceans, waterways and soils of the world. Living diatoms make up a significant portion of the Earth's biomass: they generate about 20 to 50 percent of the oxygen produced on the planet each year,[10][11] take in over 6.7 billion metric tons of silicon each year from the waters in which they live,[12] and constitute nearly half of the organic material found in the oceans. The shells of dead diatoms can reach as much as a half-mile (800 m) deep on the ocean floor, and the entire Amazon basin is fertilized annually by 27 million tons of diatom shell dust transported by transatlantic winds from the African Sahara, much of it from the Bodélé Depression, which was once made up of a system of fresh-water lakes.[13][14]
https://en.wikipedia.org/wiki/Diatom
Plasmodesmata (singular: plasmodesma) are microscopic channels which traverse the cell walls of plant cells[2] and some algal cells, enabling transport and communication between them. Plasmodesmata evolved independently in several lineages,[3] and species that have these structures include members of the Charophyceae, Charales, Coleochaetales and Phaeophyceae (which are all algae), as well as all embryophytes, better known as land plants.[4]Unlike animal cells, almost every plant cell is surrounded by a polysaccharide cell wall. Neighbouring plant cells are therefore separated by a pair of cell walls and the intervening middle lamella, forming an extracellular domain known as the apoplast. Although cell walls are permeable to small soluble proteins and other solutes, plasmodesmata enable direct, regulated, symplastic transport of substances between cells. There are two forms of plasmodesmata: primary plasmodesmata, which are formed during cell division, and secondary plasmodesmata, which can form between mature cells.[5]
Similar structures, called gap junctions[6] and membrane nanotubes, interconnect animal cells[7] and stromules form between plastids in plant cells.[8]
https://en.wikipedia.org/wiki/Plasmodesma
The Embryophyta (/ˌɛmbriˈɒfətə, -oʊˈfaɪtə/), or land plants, are the most familiar group of green plants that comprise vegetation on Earth. Embryophytes (/ˈɛmbriəˌfaɪts/) have a common ancestor with green algae, having emerged within the Phragmoplastophyta clade of green algae as sister of the Zygnematophyceae/Mesotaeniaceae.[12] The Embryophyta consist of the bryophytes plus the polysporangiophytes.[13] Living embryophytes therefore include hornworts, liverworts, mosses, lycophytes, ferns, gymnosperms and flowering plants. The land plants have diplobiontic life cycles and is shown that the Charophycean green algae gave rise to land plants.[14]
The embryophytes are informally called land plants because they live primarily in terrestrial habitats (with exceptional members who evolved to live once again in aquatic habitats), while the related green algae are primarily aquatic. Embryophytes are complex multicellular eukaryotes with specialized reproductive organs. The name derives from their innovative characteristic of nurturing the young embryo sporophyte during the early stages of its multicellular development within the tissues of the parent gametophyte. With very few exceptions, embryophytes obtain their energy by photosynthesis, that is by using the energy of sunlight to synthesize their food from carbon dioxide and water.
https://en.wikipedia.org/wiki/Embryophyte
The symplast of a plant is the inner side of the plasma membrane in which water and low-molecular-weight solutes can freely diffuse. Symplast cells have more than one nucleus. Symplast could also refer to the cytoplasmic connection made between cells by the plasmodesmata.
The plasmodesmata allow the direct flow of small molecules such as sugars, amino acids, and ions between cells. Larger molecules, including transcription factors and plant viruses, can also be transported through with the help of actin structures.
This allows direct cytoplasm-to-cytoplasm flow of water and other nutrients along concentration gradients. In particular, symplastic flow is used in the root systems to bring in nutrients from soil. It moves these solutes from epidermis cells through the cortex into the endodermis. Once solutes reach the endodermal cells through apoplastic flow, they are forced into the symplastic pathway due to the presence of the Casparian strip. Once the solutes are passively filtered, they eventually reach the pericycle, where it can be moved into the xylem for long-distance transport. It is contrasted with the apoplastic flow, which uses cell wall transport.[1]
https://en.wikipedia.org/wiki/Symplast
Protoplast (from Ancient Greek πρωτόπλαστος (prōtóplastos) 'first-formed'), is a biological term coined by Hanstein in 1880 to refer to the entire cell, excluding the cell wall.[1][2] Protoplasts can be generated by stripping the cell wall from plant,[3]bacterial,[4][5] or fungal cells[5][6] by mechanical, chemical or enzymatic means.
Protoplasts differ from spheroplasts in that their cell wall has been completely removed.[4][5] Spheroplasts retain part of their cell wall.[7] In the case of Gram-negative bacterial spheroplasts, for example, the peptidoglycan component of the cell wall has been removed but the outer membrane component has not.[4][5]
https://en.wikipedia.org/wiki/Protoplast
The perivitelline space is the space between the zona pellucida and the cell membrane of an oocyte or fertilized ovum.[1]In the slow block to polyspermy, the cortical granules released from the ovum are deposited in the perivitelline space. Polysaccharides released in the granules cause the space to swell, pushing the zona pellucida farther from the oocyte.[1]The hydrolytic enzymes released by the granules cause the zona reaction, which removes the ZP3 ligands from the zona pellucida.[1]
https://en.wikipedia.org/wiki/Perivitelline_space
A polar body is a small haploid cell that is formed at the same time as an egg cell during oogenesis, but generally does not have the ability to be fertilized.
When certain diploid cells in animals undergo cytokinesis after meiosis to produce egg cells, they sometimes divide unevenly. Most of the cytoplasm is segregated into one daughter cell, which becomes the egg or ovum, while the smaller polar bodiesonly get a small amount of cytoplasm. They frequently die and disintegrate by apoptosis, but in some cases remain and can be important in the life cycle of the organism.[1]
Twinning[edit]
Polar body twinning is a hypothesized form of twinning in meiosis, where one or more polar bodies do not disintegrate and are fertilized by sperm.[2]
Twinning would occur, in principle, if the egg cell and a polar body were both fertilized by separate sperms. However, even if fertilization occurs, further development would usually not occur because the zygote formed by the fusion of the sperm and polar body would not have enough cytoplasm or stored nutrients to feed the developing embryo.
Polar bodies were first reported in 1824 by Carus in gastropods, but their role was not clarified until the work of Butschli in 1875, Giard in 1876, and finally Hertwig in 1877. These structures were often confused with egg fragments or expelled yolk masses, but were eventually referred to as directional bodies (or Richtungskörper), a term implying the place where the maturation divisions start. The common names "polocytes" and "polar bodies" derive from their polar position in the eggs.[1] Polar bodies were characterized in the early 20th century, by O. Hertwig, T. Boveri, and E.L. Mark, as non-functioning egg cells which disintegrated because the spermatozoon, with rare exceptions, could not fertilize them and instead chemically triggered their dissolution.[3]
Polar bodies serve to eliminate one half of the diploid chromosome set produced by meiotic division in the egg, leaving behind a haploid cell. To produce the polar bodies, the cell must divide asymmetrically, which is fueled by furrowing (formation of a trench) near a particular point on the cell membrane. The presence of chromosomes induces the formation of an actomyosin cortical cap, a myosin II ring structure and a set of spindle fibers, the rotation of which promotes invagination at the edge of the cell membrane and splits the polar body away from the oocyte.[4]
Meiotic errors can lead to aneuploidy in the polar bodies, which, in the majority of cases, produces an aneuploid zygote. Errors can occur during either of the two meiotic divisions that produce each polar body, but are more pronounced if they occur during the formation of the first polar body, because the formation of the first polar body influences the chromosomal makeup of the second. For example, predivision (the separation of chromatids before anaphase) in the first polar body can induce the formation of an aneuploid polar body. Therefore, the formation of the first polar body is an especially important factor in forming a healthy zygote.[5]
However, chromosomally abnormal polar bodies are not guaranteed to induce the development of an abnormal zygote. A euploid zygote can be produced if the aneuploidy is reciprocal: one polar body has an extra chromosome and the other lacks the same chromosome (see also uniparental disomy). If the extra chromosome is absorbed into a polar body rather than being passed into the oocyte, trisomy can be avoided. Whether this is a chance event or is some way influenced by the microenvironment is unclear. In at least one case, this euploid zygote has been traced through development to birth as a healthy child with a normal chromosome count.[6]
Medical applications[edit]
A polar body biopsy is the sampling of a polar body of an oocyte. After sampling of a polar body, subsequent analysis can be used to predict viability and pregnancy chance of the oocyte, as well as the future health of a person resulting such pregnancy. The latter use makes it a form of preimplantation genetic screening (PGS). Compared to a blastocyst biopsy, a polar body biopsy can potentially be of lower costs, less harmful side-effects, and more sensitive in detecting abnormalities.[6] The main advantage of the use of polar bodies in PGD is that they are not necessary for successful fertilisation or normal embryonic development, thus ensuring no deleterious effect for the embryo.
One of the disadvantages of PB biopsy is that it only provides information about the maternal contribution to the embryo, which is why cases of autosomal dominant and X-linked disorders that are maternally transmitted can be diagnosed, and autosomal recessive disorders can only partially be diagnosed. Another drawback is the increased risk of diagnostic error, for instance due to the degradation of the genetic material or events of recombination that lead to heterozygous first polar bodies.
Parthenogenesis[edit]
In some species, the polar body may re-merge with the egg cell. This can result in a viable embryo that has only one parent, a process called parthenogenesis.[7]
Additional images[edit]
https://en.wikipedia.org/wiki/Polar_body
Category:Germ cells

CL:0000039
Subcategories
This category has the following 4 subcategories, out of 4 total.
G
- Germ line cells (3 P)
N
- Germ cell neoplasia (21 P)
S
- Spermicide (6 P)
- Germ cell structures (6 P)
Pages in category "Germ cells"
The following 38 pages are in this category, out of 38 total. This list may not reflect recent changes (learn more).
https://en.wikipedia.org/wiki/Category:Germ_cells
Capacitation is the penultimate[1] step in the maturation of mammalian spermatozoa and is required to render them competent to fertilize an oocyte.[2] This step is a biochemical event; the sperm move normally and look mature prior to capacitation. In vivo, capacitation occurs after ejaculation, when the spermatozoa leave the vagina and enter the superior female reproductive tract. The uterus aids in the steps of capacitation by secreting sterol-binding albumin, lipoproteins, and proteolyticand glycosidasic enzymes such as heparin.
For purposes of in vitro fertilization, capacitation occurs by incubating spermatozoa that have either undergone ejaculation or have been extracted from the epididymisand incubated in a defined medium for several hours. There are different techniques to perform the capacitation step: simple washing, migration (swim-up), density gradients, and filter. The objective is to isolate as many motile spermatozoa as possible and to eliminate non-motile or dead spermatozoa. After either in vivo or in vitrocapacitation the sperm must undergo the final maturation step, activation, involving the acrosome reaction.
Non-mammalian spermatozoa do not require this capacitation step and are ready to fertilize an oocyte immediately after release from the male.
https://en.wikipedia.org/wiki/Capacitation
During fertilization, a sperm must first fuse with the plasma membrane and then penetrate the female egg cell to fertilize it. Fusing to the egg cell usually causes little problem, whereas penetrating through the egg's hard shell or extracellular matrix can be more difficult. Therefore, sperm cells go through a process known as the acrosome reaction, which is the reaction that occurs in the acrosome of the sperm as it approaches the egg. The acrosome is a cap-like structure over the anterior half of the sperm's head.
As the sperm approaches the zona pellucida of the egg, which is necessary for initiating the acrosome reaction, the membrane surrounding the acrosome fuses with the plasma membrane of the sperm's head, exposing the contents of the acrosome. The contents include surface antigens necessary for binding to the egg's cell membrane, and numerous enzymes which are responsible for breaking through the egg's tough coating and allowing fertilization to occur.[1]
Variations among species[edit]
There are considerable species variations in the morphology and consequences of the acrosome reaction. In several species, the trigger for the acrosome reaction has been identified in a layer that surrounds the egg.
Echinoderms[edit]
In some lower animal species, a protuberance (the acrosomal process) forms at the apex of the sperm head, supported by a core of actin microfilaments. The membrane at the tip of the acrosomal process fuses with the egg's plasma membrane.
In some echinoderms, including starfish and sea urchins, a significant portion of the exposed acrosomal content contains a protein that temporarily holds the sperm on the egg's surface.
Mammals[edit]
In mammals, the acrosome reaction releases hyaluronidase and acrosin; their role in fertilization is not yet clear. The acrosomal reaction does not begin until the sperm comes into contact with the oocyte's zona pellucida. Upon coming into contact with the zona pellucida, the acrosomal enzymes begin to dissolve, and the actin filament comes into contact with the zona pellucida. Once the two meet, a calcium influx occurs, causing a signaling cascade. The cortical granules inside the oocyte then fuse to the outer membrane, and a quick fast block reaction occurs.
It also alters a patch of pre-existing sperm plasma membrane so that it can fuse with the egg plasma membrane.
A sperm penetration assay includes an acrosome reaction test that assesses how well a sperm can perform during the fertilization process. Sperm that are unable to go through the acrosome reaction properly will not be able to fertilize an egg. However, this problem only occurs in about 5% of men that have the test done. This test is rather expensive and provides limited information on a man's fertility.[2]
In other cases, such as in the wood mouse Apodemus sylvaticus, premature acrosome reactions have been found to cause increased motility in aggregates of spermatozoa promoting fertilization.[3]
The process[edit]
The acrosomal reaction usually takes place in the ampulla of the fallopian tube (site of fertilization) when the sperm penetrates the secondary oocyte. A few events precede the actual acrosome reaction. The sperm cell acquires a "hyperactive motility pattern" by which its flagellum produces vigorous whip-like movements that propel the sperm through the cervical canal and uterine cavity until it reaches the isthmus of the fallopian tube. The sperm approaches the ovum in the ampulla of the fallopian tube with the help of various mechanisms, including chemotaxis. Glycoproteins on the outer surface of the sperm then bind with glycoproteins on the zona pellucida of the ovum.
Sperm that did not initiate the acrosome reaction prior to reaching to the zona pellucida are unable to penetrate the zona pellucida. Since the acrosome reaction has already occurred, sperm are then able to penetrate the zona pellucida due to mechanical action of the tail, not because of the acrosome reaction itself.[4]
The first stage is the penetration of corona radiata, by releasing hyaluronidase from the acrosome to digest cumulus cells surrounding the oocyte and exposing acrosin attached to the inner membrane of the sperm. The cumulus cells are embedded in a gel-like substance made primarily of hyaluronic acid, and developed in the ovary with the egg and support it as it grows. The acrosome reaction must occur before the sperm cell reaches the zona pellucida.[4]
Acrosin digests the zona pellucida and membrane of the oocyte. Part of the sperm's cell membrane then fuses with the egg cell's membrane, and the contents of the head sink into the egg. In the mouse, it has been demonstrated that ZP3, one of the proteins that make up the zona pellucida, binds to a partner molecule (to the β1,4-galactosyl transferase receptors) on the sperm. This lock-and-key type mechanism is species-specific and prevents the sperm and egg of different species from fusing. The zona pellucida also releases Ca granules to prevent other sperm from binding. There is some evidence that this binding is what triggers the acrosome to release the enzymes that allow the sperm to fuse with the egg. A similar mechanism likely occurs in other mammals, but the diversity of zona proteins across species means that the relevant protein and receptor may differ.
More recent scientific evidence demonstrates that the acrosomal reaction is necessary to expose a protein called IZUMO1 on the sperm: without the reaction, sperm can still penetrate through the zona pellucida to the egg membrane, but cannot fuse.[5] As seen in mouse studies, IZUMO1 binds to the oocyte protein JUNO and once bound together, the sperm and the egg fuse together to form two pronuclei.[6][7] These pronuclei supply the zygote with the genetic material necessary for the formation of an embryo. Additionally, once the fusion of the sperm and oocyte is complete, phospholipase C zeta is released from the sperm.
Upon penetration, if all is normally occurring, the process of egg-activation occurs, and the oocyte is said to have become activated. This is thought to be induced by a specific protein phospholipase c zeta. It undergoes its secondary meiotic division, and the two haploid nuclei (paternal and maternal) fuse to form a zygote. To prevent polyspermy and minimize the possibility of producing a triploid zygote, several changes to the egg's cell membranes render them impenetrable shortly after the first sperm enters the egg (such as the rapid loss of JUNO).[7]
Spontaneous acrosome reaction[edit]
Spermatozoa can initiate the acrosomal reaction well in advance of reaching the zona pellucida, as well as in vitro in an appropriate culture medium. This is referred to as spontaneous acrosome reaction (SAR).
It is now known that in a certain sense, this phenomenon is physiologically normal across mammalian species. The acrosome reaction is induced by passage through the cumulus oophorus cells, mediated by the hormones they secrete (such as progesterone, LPA, LPC).[5][8][9]
However, the physiological role of truly spontaneous acrosomal reaction, occurring well before this point in the female reproductive tract, or in vitro, is a separate phenomenon.
In mice, it has been well established as physiologically normal and common. Mouse sperm which have undergone fully spontaneous acrosome reaction are still able to fertilize eggs.[5] Furthermore, the rate of spontaneous acrosome reaction is higher in more promiscuous species such as Apodemus sylvaticus, which face a high level of sperm competition.[10]
In humans, on the other hand, it remains disputed where exactly the acrosome reaction is initiated in physiological fertilization, due to experimental constraints (for example, animal studies may make use of transgenic mice with fluorescent sperm, while human studies cannot).[9]
Studies have been done with the intent of linking in vitro SAR rate in human sperm to sperm quality and fertilization rate, but the overall results are mixed, and do not seem to be clinically useful as of 2018.[11]
In in vitro fertilization[edit]
When using intracytoplasmic sperm injection (ICSI) for IVF, the implantation rate is higher in oocytes injected with spermatozoa that have undergone acrosome reaction (~40%) vs. those injected with nonreacted spermatozoa (~10%). The implantation rate is ~25% in when injected with both reacted and nonreacted spermatozoa. The delivery rate per cycle follows the same trend.[12]
The acrosome reaction can be stimulated in vitro by substances a sperm cell may encounter naturally, such as progesterone or follicular fluid, as well as the more commonly used calcium ionophore A23187.
Assessment[edit]
Birefringence microscopy,[12] flow cytometry[13] or fluorescence microscopy can be used for assessing the shedding of the acrosome or "acrosome reaction" of a sperm sample. Flow cytometry and fluorescence microscopy are usually done after staining with a fluoresceinated lectin such as FITC-PNA, FITC-PSA, FITC-ConA, or fluoresceinated antibody such as FITC-CD46.[14] The antibodies/lectins have a high specificity for different parts of the acrosomal region, and will only bind to a specific site (acrosomal content/ inner/outer membrane). If bound to a fluorescent molecule, regions where these probes have bound can be visualised. Sperm cells with artificially induced acrosome reactions may serve as positive controls.
For fluorescence microscopy, a smear of washed sperm cells is made, air-dried, permeabilized, and then stained. Such a slide is then viewed under the light of a wavelength that will cause the probe to fluoresce if it is bound to the acrosomal region. At least 200 cells are considered arbitrarily and classified as either acrosome intact (fluorescing bright green), or acrosome reacted (no probe present, or only on the equatorial region). It is then expressed as a percentage of the counted cells.
For assessment with flow cytometry, the washed cells are incubated with the chosen probe, possibly passed again, then sampled in a flow cytometer. After gating the cell population according to forward- and side-scatter, the resulting data can be analyzed (E.g. mean fluorescences compared). With this technique, a probe for viability such as propidium iodide (PI) could also be included in order to exclude dead cells from the acrosome assessment, since many sperm cells will spontaneously lose their acrosome when they die.
See also[edit]
https://en.wikipedia.org/wiki/Acrosome_reaction
Subcategories
This category has the following 14 subcategories, out of 14 total.
A
- Andrology journals (4 P)
C
D
- Deaths from prostate cancer (706 P)
E
I
M
T
- Deaths from testicular cancer (31 P)
Pages in category "Andrology"
The following 54 pages are in this category, out of 54 total. This list may not reflect recent changes (learn more).
M
https://en.wikipedia.org/wiki/Category:Andrology
No comments:
Post a Comment