Paraquat (trivial name; ), or N,N′-dimethyl-4,4′-bipyridinium dichloride (systematic name), also known as methyl viologen, is an organic compound with the chemical formula [(C6H7N)2]Cl2. It is classified as a viologen, a family of redox-active heterocycles of similar structure.[5] This salt is one of the most widely used herbicides. It is quick-acting and non-selective, killing green plant tissue on contact. It is also toxic to human beings and animals due to its redox activity, which produces superoxide anions. It has been linked to the development of Parkinson's disease[6][7] and is banned in several countries.
Paraquat may be in the form of salt with chloride or other anions; quantities of the substance are sometimes expressed by cation mass alone (paraquat cation, paraquat ion).
The name is derived from the para positions of the quaternary nitrogens.
https://en.wikipedia.org/wiki/Paraquat
Glyphosate (IUPAC name: N-(phosphonomethyl)glycine) is a broad-spectrum systemic herbicide and crop desiccant. It is an organophosphorus compound, specifically a phosphonate, which acts by inhibiting the plant enzyme 5-enolpyruvylshikimate-3-phosphate synthase. It is used to kill weeds, especially annual broadleaf weeds and grasses that compete with crops. Its herbicidal effectiveness was discovered by Monsanto chemist John E. Franz in 1970. Monsanto brought it to market for agricultural use in 1974 under the trade name Roundup. Monsanto's last commercially relevant United States patent expired in 2000.
https://en.wikipedia.org/wiki/Glyphosate
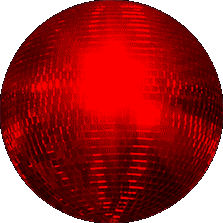

Canisters are commonly filled with
silica gel and other
molecular sieves used as desiccant in drug containers to keep contents dry.
https://en.wikipedia.org/wiki/Desiccant

Hygroscopy is the phenomenon of attracting and holding water molecules via either absorption or adsorption from the surrounding environment, which is usually at normal or room temperature. If water molecules become suspended among the substance's molecules, adsorbing substances can become physically changed, e.g., changing in volume, boiling point, viscosity or some other physical characteristic or property of the substance.
https://en.wikipedia.org/wiki/Hygroscopy
https://en.wikipedia.org/wiki/nuclear_reactor
https://en.wikipedia.org/wiki/Absorption_(chemistry)
https://en.wikipedia.org/wiki/Adsorption
https://en.wikipedia.org/wiki/Sulfuric_acid
https://en.wikipedia.org/wiki/Hygroscopy
https://en.wikipedia.org/wiki/Methanol
https://en.wikipedia.org/wiki/Potassium_hydroxide
https://en.wikipedia.org/wiki/Calcium_chloride
https://en.wikipedia.org/wiki/Humectant
https://en.wikipedia.org/wiki/Salt_(chemistry)
https://en.wikipedia.org/wiki/Hygroscopy#Deliquescence
https://en.wikipedia.org/wiki/Atmosphere
https://en.wikipedia.org/wiki/Composite_material
https://en.wikipedia.org/wiki/Bimetallic_strip
https://en.wikipedia.org/wiki/Capillary_action
https://en.wikipedia.org/wiki/Vapor_pressure
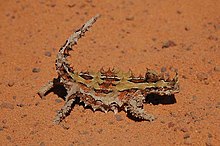
The
thorny dragon features hygroscopic grooves between the spines of its skin to capture water in its desert habitat.
https://en.wikipedia.org/wiki/Hygroscopy
https://en.wikipedia.org/wiki/Capillary_action
https://en.wikipedia.org/wiki/Surface_tension
https://en.wikipedia.org/wiki/Carbon-fiber-reinforced_polymers
https://en.wikipedia.org/wiki/Category:Chemical_properties
https://en.wikipedia.org/wiki/Hydrophile
https://en.wikipedia.org/wiki/Hydrophobe
https://en.wikipedia.org/wiki/Equilibrium_moisture_content

In chemistry, efflorescence (which means "to flower out" in French) is the migration of a salt to the surface of a porous material, where it forms a coating. The essential process involves the dissolving of an internally held salt in water, or occasionally in another solvent. The water, with the salt now held in solution, migrates to the surface, then evaporates, leaving a coating of the salt.
In what has been described as "primary efflorescence", the water is the invader and the salt was already present internally, and a reverse process, where the salt is originally present externally and is then carried inside in solution, is referred to as "secondary efflorescence".
Efflorescences can occur in natural and built environments. On porous construction materials it may present a cosmetic outer problem only (primary efflorescence causing staining), but can sometimes indicate internal structural weakness (migration/degradation of component materials). Efflorescence may clog the pores of porous materials, resulting in the destruction of those materials by internal water pressure, as seen in the spalling of brick.
Examples[edit]
- A 5 molar concentration aqueous droplet of NaCl will spontaneously crystallize at 45% relative humidity (298 K) to form an NaCl cube by the mechanism of homogeneous nucleation. The original water is released to the gas phase.
- Gypsum (CaSO4.2H2O) is a hydrate solid that, in a sufficiently dry environment, will give up its water to the gas phase and form anhydrite(CaSO4).
- Copper(II) sulfate (bluestone) (CuSO4.5H2O) is a blue crystalline solid that when exposed to air, slowly loses water of crystallization from its surface to form a white layer of anhydrous copper(II) sulfate.
- Sodium carbonate deca hydrate (Na2CO3.10H2O) will lose water when exposed to air.
Masonry[edit]
Primary efflorescence[edit]
Primary efflorescence is named such, as it typically occurs during the initial cure of a cementitious product. It often occurs on masonry construction, particularly brick, as well as some firestop mortars, when water moving through a wall or other structure, or water being driven out as a result of the heat of hydration as cement stone is being formed, brings salts to the surface that are not commonly bound as part of the cement stone. As the water evaporates, it leaves the salt behind, which forms a white, fluffy deposit, that can normally be brushed off. The resulting white deposits are referred to as "efflorescence" in this instance. In this context efflorescence is sometimes referred to as "saltpetering." Since primary efflorescence brings out salts that are not ordinarily part of the cement stone, it is not a structural, but, rather, an aesthetic concern.[citation needed]
For controlling primary efflorescence, formulations containing liquid fatty acid mixtures (e.g., oleic acid and linoleic acid) have commonly been used. The oily liquid admixture is introduced into the batch mix at an early stage by coating onto the sand particles prior to the introduction of any mix water, so that the oily admixture is distributed uniformly throughout the concrete batch mix.[1]
Secondary efflorescence[edit]
Secondary efflorescence is named such as it does not occur as a result of the forming of the cement stone or its accompanying hydration products. Rather, it is usually due to the external influence of concrete poisons, such as chlorides. A very common example of where secondary efflorescence occurs is steel-reinforced concrete bridges as well as parking garages. Saline solutions are formed due to the presence of road salt in the winter. This saline solution is absorbed into the concrete, where it can begin to dissolve cement stone, which is of primary structural importance. Virtual stalactitescan be formed in some cases as a result of dissolved cement stone, hanging off cracks in concrete structures. Where this process has taken hold, the structural integrity of a concrete element is at risk. This is a common traffic infrastructure and building maintenance concern. Secondary efflorescence is akin to osteoporosis of the concrete.
For controlling secondary efflorescence, admixtures containing aqueous-based calcium stearate dispersion (CSD) are often added at a later stage of the batching process with the mix water. In a typical batching process, sand is first charged into the mixer, then the oil-based primary anti-efflorescence admixture is added with constant mixing to allow the oil to coat the sand. Then coarse aggregates, colorants, and cement are added, followed by water. If CSD is used, it is then introduced usually at this point during or after the addition of the mix water. CSD is an aqueous dispersion wherein fine solid particles of calcium stearate are suspended in the water uniformly. Commercially available CSD has an average particle size of about 1 to 10 micrometres. The uniform distribution of CSD in the mix may render the resulting concrete masonry unit water repellent, as CSD particles are well distributed in the pores of the unit to interfere with the capillary movement of water.[1]
Calthemite is also a secondary deposit derived from concrete, mortar or lime, which can be mistakenly assumed to be efflorescence. Calthemites are usually deposited as calcite which is the most stable polymorph of calcium carbonate (CaCO3).[2][3]
Protecting against efflorescence[edit]
The only way to completely and permanently prevent (both primary and secondary) efflorescence in cementitious materials is by using special admixtures that chemically react with and bind the salt-based impurities in the concrete when hydrogen (H) is present. The chemical reaction in these special additives fuses the sodium chloride on a nanomolecular level, converting it into non-sodium chemicals and other harmless matter that will not leach out or migrate to the surface. In fact, the nanotechnology in these additives can be up to 100,000 times smaller than even the smallest cement particles, allowing their molecules to literally pass through cement minerals or sand particles and ultimately become part of the cement or sand with which they react. And since they require the presence of hydrogen they stop reacting as the concrete dries out and begin reacting again when the concrete is exposed to moisture.
It is also possible to protect porous building materials, such as brick, tiles, concrete and purely against efflorescence by treating the material with an impregnating, hydro-phobic sealer. This is a sealer that repels water and will penetrate deeply enough into the material to keep water and dissolved salts well away from the surface. However, in climates where freezing is a concern, such a sealer may lead to damage from freeze/thaw cycles. And while it will help to protect against efflorescence, it cannot permanently prevent the problem.
Efflorescence can often be removed from concrete using phosphoric acid. After application the acid dilution is neutralised with mild diluted detergent, and then well rinsed with water. However, if the source of the water penetration is not addressed efflorescence may reappear.
Common rebar protective measures include the use of epoxy coating as well as the use of a slight electrical charge, both of which prevent rusting. One may also use stainless steel rebar.
Certain cement types are less resistant to chlorides than others. The choice of cement, therefore, can have a large effect upon the concrete's reaction to chlorides.
Today's water repellents help create a vapor permeable barrier; liquid water, especially from wind driven rains, will stay out of the brick and masonry. Water vapor from the interior of the building, or from the underside of pavers can escape. This will reduce efflorescence, spalling and scaling that can occur from water being trapped inside the brick substrate and freezing during cold weather. Years ago, the water repellents trapped moisture in the masonry wall creating more problems than they solved. Condensation in areas that experienced the four seasons were much more problematic than their counterparts.
Image gallery[edit]
Primary efflorescence on a brick wall in Germany.
Secondary efflorescence - dissolving the cement stone and attacking rebar
Concrete derived secondary deposit of calcium carbonate creating calthemite stalactites, which can be mistakenly confused with efflorescence.
See also[edit]
https://en.wikipedia.org/wiki/Efflorescence

Anhydrite, or anhydrous calcium sulfate, is a mineral with the chemical formula CaSO4. It is in the orthorhombic crystal system, with three directions of perfect cleavage parallel to the three planes of symmetry. It is not isomorphous with the orthorhombic barium (baryte) and strontium (celestine) sulfates, as might be expected from the chemical formulas. Distinctly developed crystals are somewhat rare, the mineral usually presenting the form of cleavage masses. The Mohs hardness is 3.5, and the specific gravity is 2.9. The color is white, sometimes greyish, bluish, or purple. On the best developed of the three cleavages, the lustre is pearly; on other surfaces it is glassy. When exposed to water, anhydrite readily transforms to the more commonly occurring gypsum, (CaSO4·2H2O) by the absorption of water. This transformation is reversible, with gypsum or calcium sulfate hemihydrate forming anhydrite by heating to around 200 °C (400 °F) under normal atmospheric conditions.[5] Anhydrite is commonly associated with calcite, halite, and sulfides such as galena, chalcopyrite, molybdenite, and pyrite in vein deposits.
https://en.wikipedia.org/wiki/Anhydrite

Sodium chloride ,[8] commonly known as salt (although sea salt also contains other chemical salts), is an ionic compound with the chemical formula NaCl, representing a 1:1 ratio of sodiumand chloride ions. With molar masses of 22.99 and 35.45 g/mol respectively, 100 g of NaCl contains 39.34 g Na and 60.66 g Cl. Sodium chloride is the salt most responsible for the salinity of seawater and of the extracellular fluid of many multicellular organisms. In its edible form of table salt, it is commonly used as a condiment and food preservative. Large quantities of sodium chloride are used in many industrial processes, and it is a major source of sodium and chlorine compounds used as feedstocks for further chemical syntheses. A second major application of sodium chloride is de-icing of roadways in sub-freezing weather.
https://en.wikipedia.org/wiki/Sodium_chloride

Thrust-to-weight ratio is a dimensionless ratio of thrust to weight of a rocket, jet engine, propeller engine, or a vehicle propelled by such an engine that is an indicator of the performance of the engine or vehicle.
The instantaneous thrust-to-weight ratio of a vehicle varies continually during operation due to progressive consumption of fuel or propellant and in some cases a gravity gradient. The thrust-to-weight ratio based on initial thrust and weight is often published and used as a figure of merit for quantitative comparison of a vehicle's initial performance.
Dry Thrust
https://en.wikipedia.org/wiki/Thrust-to-weight_ratio

A propellant (or propellent) is a mass that is expelled from a vehicle, such as a rocket, in such a way as to create a thrust in accordance with Newton's third law of motion, and "propel" the vehicle forward. The engine that expels the propellant is called a reaction engine. Although the term "propellant" is often used in chemical rocket design to describe a combined fuel/propellant, propellants should not be confused with the fuel that is used by an engine to produce the energy that expels the propellant. Even though the byproducts of substances used as fuel are also often used as a reaction mass to create the thrust, such as with a chemical rocket engine, propellant and fuel are two distinct concepts.
In electrically powered spacecraft, electricity is used to accelerate the propellant. An electrostatic force may be used to expel positive ions, or the Lorentz force may be used to expel negative ions and electrons as the propellant. Electothermal engines use the electromagnetic force to heat low molecular weight gases (e.g. hydrogen, helium, ammonia) into a plasma and expel the plasma as propellant. In the case of a resistojet rocket engine, the compressed propellant is simply heated using resistive heating as it is expelled to create more thrust.
In chemical rockets and aircraft, fuels are used to produce an energetic gas that can be directed through a nozzle, thereby producing thrust. In rockets, the burning of rocket fuel produces an exhaust, and the exhausted material is usually expelled as a propellant under pressure through a nozzle. The exhaust material may be a gas, liquid, plasma, or a solid. In powered aircraft without propellers such as jets, the propellant is usually the product of the burning of fuel with atmospheric oxygen so that the resulting propellant product has more mass than the fuel carried on the vehicle.
The propellant or fuel may also simply be a compressed fluid, with the potential energy that is stored in the compressed fluid used to expel the fluid as the propellant. The energy stored in the fluid was added to the system when the fluid was compressed, such as compressed air. The energy applied to the pump or thermal system that is used to compress the air is stored until it is released by allowing the propellant to escape. Compressed fluid may also be used only as energy storage along with some other substance as the propellant, such as with a water rocket, where the energy stored in the compressed air is the fuel and the water is the propellant.
Proposed photon rockets would use the relativistic momentum of photons to create thrust. Even though photons do not have mass, they can still act as a propellant because they move at relativistic speed, i.e., the speed of light. In this case Newton's third Law of Motion is inadequate to model the physics involved and relativistic physics must be used.
In chemical rockets, chemical reactions are used to produce energy which creates movement of a fluid which is used to expel the products of that chemical reaction (and sometimes other substances) as propellants. For example, in a simple hydrogen/oxygen engine, hydrogen is burned (oxidized) to create H2O and the energy from the chemical reaction is used to expel the water (steam) to provide thrust. Often in chemical rocket engines, a higher molecular mass substance is included in the fuel to provide more reaction mass.
Rocket propellant may be expelled through an expansion nozzle as a cold gas, that is, without energetic mixing and combustion, to provide small changes in velocity to spacecraft by the use of cold gas thrusters, usually as maneuvering thrusters.
To attain a useful density for storage, most propellants are stored as either a solid or a liquid.
https://en.wikipedia.org/wiki/Propellant

An ion thruster, ion drive, or ion engine is a form of electric propulsion used for spacecraft propulsion. It creates thrust by accelerating ions using electricity.
An ion thruster ionizes a neutral gas by extracting some electrons out of atoms, creating a cloud of positive ions. These ion thrusters rely mainly on electrostatics as ions are accelerated by the Coulomb force along an electric field. Temporarily stored electrons are finally reinjected by a neutralizer in the cloud of ions after it has passed through the electrostatic grid, so the gas becomes neutral again and can freely disperse in space without any further electrical interaction with the thruster. In contrast, electromagnetic thrusters use the Lorentz force to accelerate all species (free electrons as well as positive and negative ions) in the same direction whatever their electric charge, and are specifically referred to as plasma propulsion engines, where the electric field is not in the direction of the acceleration.[1][2]
Ion thrusters in operational use typically consume 1–7 kW of power, have exhaust velocities around 20–50 km/s (Isp 2000–5000 s), and possess thrusts of 25–250 mN and a propulsive efficiency 65–80%.[3][4] though experimental versions have achieved 100 kW (130 hp), 5 N (1.1 lbf).[5]
The Deep Space 1 spacecraft, powered by an ion thruster, changed velocity by 4.3 km/s (2.7 mi/s) while consuming less than 74 kg (163 lb) of xenon. The Dawn spacecraft broke the record, with a velocity change of 11.5 km/s (41,000 km/h), though it was only half as efficient, requiring 425 kg (937 lb) of xenon.[6]
Applications include control of the orientation and position of orbiting satellites (some satellites have dozens of low-power ion thrusters) and use as a main propulsion engine for low-mass robotic space vehicles (such as Deep Space 1 and Dawn).[3][4]
Ion thrust engines are practical only in the vacuum of space and cannot take vehicles through the atmosphere because ion engines do not work in the presence of ions outside the engine; additionally, the engine's minuscule thrust cannot overcome any significant air resistance. Moreover, notwithstanding the presence of an atmosphere (or lack thereof) an ion engine cannot generate sufficient thrust to achieve initial liftoff from any celestial body with significant surface gravity. For these reasons, spacecraft must rely on conventional chemical rockets to reach their initial orbit.
Electromagnetic thrusters[edit]
Pulsed inductive thrusters[edit]
Pulsed inductive thrusters (PIT) use pulses instead of continuous thrust and have the ability to run on power levels on the order of megawatts (MW). PITs consist of a large coil encircling a cone shaped tube that emits the propellant gas. Ammonia is the gas commonly used. For each pulse, a large charge builds up in a group of capacitors behind the coil and is then released. This creates a current that moves circularly in the direction of jθ. The current then creates a magnetic field in the outward radial direction (Br), which then creates a current in the gas that has just been released in the opposite direction of the original current. This opposite current ionizes the ammonia. The positively charged ions are accelerated away from the engine due to the electric field jθ crossing the magnetic field Br, due to the Lorentz Force.[30]
Magnetoplasmadynamic thruster[edit]
Magnetoplasmadynamic (MPD) thrusters and lithium Lorentz force accelerator (LiLFA) thrusters use roughly the same idea. The LiLFA thruster builds on the MPD thruster. Hydrogen, argon, ammonia and nitrogen can be used as propellant. In a certain configuration, the ambient gas in low Earth orbit(LEO) can be used as a propellant. The gas enters the main chamber where it is ionized into plasma by the electric field between the anode and the cathode. This plasma then conducts electricity between the anode and the cathode, closing the circuit. This new current creates a magnetic field around the cathode, which crosses with the electric field, thereby accelerating the plasma due to the Lorentz force.
The LiLFA thruster uses the same general idea as the MPD thruster, with two main differences. First, the LiLFA uses lithium vapor, which can be stored as a solid. The other difference is that the single cathode is replaced by multiple, smaller cathode rods packed into a hollow cathode tube. MPD cathodes are easily corroded due to constant contact with the plasma. In the LiLFA thruster, the lithium vapor is injected into the hollow cathode and is not ionized to its plasma form/corrode the cathode rods until it exits the tube. The plasma is then accelerated using the same Lorentz force.[31][32][33]
In 2013, Russian company the Chemical Automatics Design Bureau successfully conducted a bench test of their MPD engine for long-distance space travel.[34]
Electrodeless plasma thrusters[edit]
Electrodeless plasma thrusters have two unique features: the removal of the anode and cathode electrodes and the ability to throttle the engine. The removal of the electrodes eliminates erosion, which limits lifetime on other ion engines. Neutral gas is first ionized by electromagnetic waves and then transferred to another chamber where it is accelerated by an oscillating electric and magnetic field, also known as the ponderomotive force. This separation of the ionization and acceleration stages allows throttling of propellant flow, which then changes the thrust magnitude and specific impulse values.[35]
Helicon double layer thrusters[edit]
A helicon double layer thruster is a type of plasma thruster that ejects high velocity ionized gas to provide thrust. In this design, gas is injected into a tubular chamber (the source tube) with one open end. Radio frequency AC power (at 13.56 MHz in the prototype design) is coupled into a specially shaped antenna wrapped around the chamber. The electromagnetic wave emitted by the antenna causes the gas to break down and form a plasma. The antenna then excites a helicon wave in the plasma, which further heats it. The device has a roughly constant magnetic field in the source tube (supplied by solenoids in the prototype), but the magnetic field diverges and rapidly decreases in magnitude away from the source region and might be thought of as a kind of magnetic nozzle. In operation, a sharp boundary separates the high density plasma inside the source region and the low density plasma in the exhaust, which is associated with a sharp change in electrical potential. Plasma properties change rapidly across this boundary, which is known as a current-free electric double layer. The electrical potential is much higher inside the source region than in the exhaust and this serves both to confine most of the electrons and to accelerate the ions away from the source region. Enough electrons escape the source region to ensure that the plasma in the exhaust is neutral overall.
Variable Specific Impulse Magnetoplasma Rocket (VASIMR)[edit]
The proposed Variable Specific Impulse Magnetoplasma Rocket (VASIMR) functions by using radio waves to ionize a propellant into a plasma and then using magnetic field to accelerate the plasma out of the back of the rocket engine to generate thrust. The VASIMR is currently being developed by Ad Astra Rocket Company, headquartered in Houston, Texas, with help from Canada-based Nautel, producing the 200 kW RF generators for ionizing propellant. Some of the components and "plasma shoots" experiments are tested in a laboratory settled in Liberia, Costa Rica. This project is led by former NASA astronaut Dr. Franklin Chang-Díaz (CRC-USA). A 200 kW VASIMR test engine was in discussion to be fitted in the exterior of the International Space Station, as part of the plan to test the VASIMR in space – however plans for this test onboard ISS were canceled in 2015 by NASA, with a free flying VASIMR test being discussed by Ad Astra instead.[36] An envisioned 200 megawatt engine could reduce the duration of flight from Earth to Jupiter or Saturn from six years to fourteen months, and Mars from 7 months to 39 days.[37]
Microwave electrothermal thrusters[edit]
Under a research grant from the NASA Lewis Research Centerduring the 1980s and 1990s, Martin C. Hawley and Jes Asmussen led a team of engineers in developing a Microwave Electrothermal Thruster (MET).[38]
In the discharge chamber, microwave (MW) energy flows into the center containing a high level of ions (I), causing neutral species in the gaseous propellant to ionize. Excited species flow out (FES) through the low ion region (II) to a neutral region (III) where the ions complete their recombination, replaced with the flow of neutral species (FNS) towards the center. Meanwhile, energy is lost to the chamber walls through heat conduction and convection (HCC), along with radiation (Rad). The remaining energy absorbed into the gaseous propellant is converted into thrust.
Radioisotope thruster[edit]
A theoretical propulsion system has been proposed, based on alpha particles (He2+
or 4
2He2+
indicating a helium ion with a +2 charge) emitted from a radioisotope uni-directionally through a hole in its chamber. A neutralising electron gun would produce a tiny amount of thrust with high specific impulse in the order of millions of seconds due to the high relativistic speed of alpha particles.[39]
A variant of this uses a graphite-based grid with a static DC high voltage to increase thrust as graphite has high transparency to alpha particles if it is also irradiated with short wave UV light at the correct wavelength from a solid state emitter. It also permits lower energy and longer half life sources which would be advantageous for a space application. Helium backfill has also been suggested as a way to increase electron mean free path.
Propellants[edit]
Ionization energy represents a large percentage of the energy needed to run ion drives. The ideal propellant is thus easy to ionize and has a high mass/ionization energy ratio. In addition, the propellant should not erode the thruster to any great degree to permit long life; and should not contaminate the vehicle.[72]
Many current designs use xenon gas, as it is easy to ionize, has a reasonably high atomic number, is inert and causes low erosion. However, xenon is globally in short supply and expensive.
Some older ion thruster designs used mercury propellant. However, mercury is toxic, tended to contaminate spacecraft, and was difficult to feed accurately. A modern commercial prototype may be using mercury successfully.[73]
Other propellants, such as bismuth and iodine, show promise both for gridless designs such as Hall effect thrusters,[53][54][55] and gridded ion thrusters.[74]
For the first time in space, Iodine was used as a propellant for electric propulsion on the NPT30-I2 gridded ion thruster by ThrustMe, onboard the Beihangkongshi-1 mission launched in November 2020.[75][76][77] The CubeSat Ambipolar Thruster (CAT) used on the Mars Array of Ionospheric Research Satellites Using the CubeSat Ambipolar Thruster (MARS-CAT) mission also proposes to use solid iodine as the propellant to minimize storage volume.[62][63]
VASIMR design (and other plasma-based engines) are theoretically able to use practically any material for propellant. However, in current tests the most practical propellant is argon, which is relatively abundant and inexpensive.
Krypton is used to fuel the Hall effect thrusters aboard Starlink internet satellites, in part due to its lower cost than conventional xenon propellant.[78]
Energy efficiency[edit]

Plot of
instantaneous propulsive efficiency and
overall efficiency for a vehicle accelerating from rest as percentages of the engine efficiency. Note that peak vehicle efficiency occurs at about 1.6 times exhaust velocity.
Ion thruster efficiency is the kinetic energy of the exhaust jet emitted per second divided by the electrical power into the device.
Overall system energy efficiency is determined by the propulsive efficiency, which depends on vehicle speed and exhaust speed. Some thrusters can vary exhaust speed in operation, but all can be designed with different exhaust speeds. At the lower end of specific impulse, Isp, the overall efficiency drops, because ionization takes up a larger percentage energy and at the high end propulsive efficiency is reduced.
Optimal efficiencies and exhaust velocities for any given mission can be calculated to give minimum overall cost.
See also[edit]
https://en.wikipedia.org/wiki/Ion_thruster

Nuclear pulse propulsion or external pulsed plasma propulsion is a hypothetical method of spacecraft propulsion that uses nuclear explosions for thrust.[1] It originated as Project Orion with support from DARPA, after a suggestion by Stanislaw Ulam in 1947.[2] Newer designs using inertial confinement fusion have been the baseline for most later designs, including Project Daedalus and Project Longshot.
https://en.wikipedia.org/wiki/Nuclear_pulse_propulsion
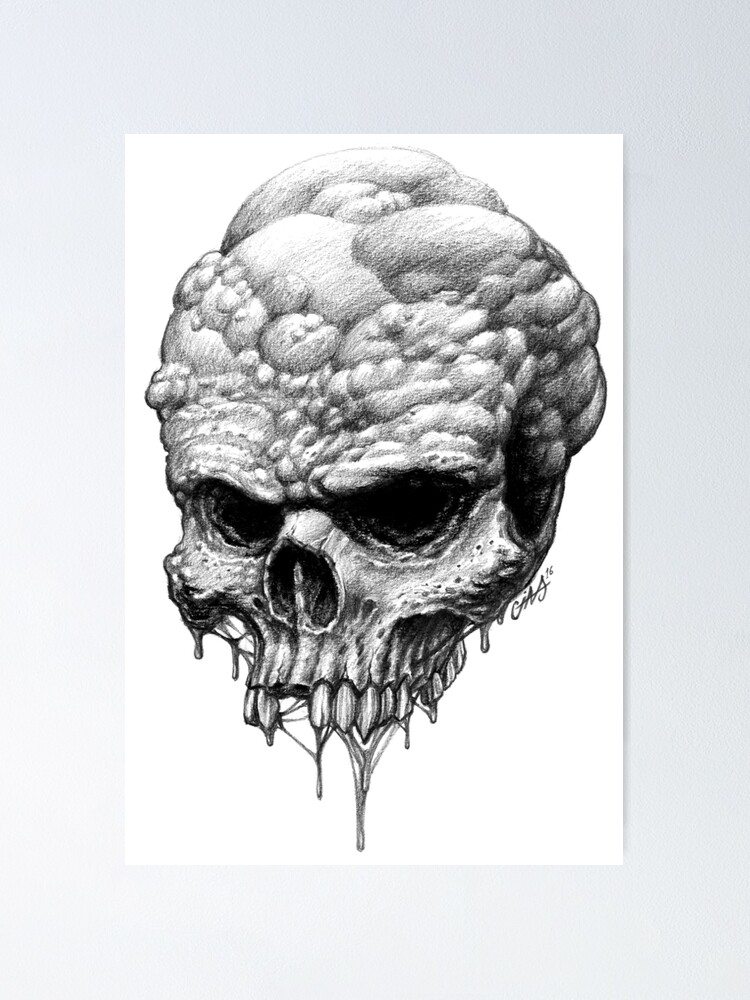
A colloid thruster (or "electrospray thruster") is a type of low thrust electric propulsion rocket engine that uses electrostatic acceleration of charged liquid droplets for propulsion. In a colloidthruster, charged liquid droplets are produced by an electrospray process and then accelerated by a static electric field. The liquid used for this application tends to be a low-volatility ionic liquid.
Like other ion thrusters, its benefits include high efficiency, thrust density, and specific impulse; however it has very low total thrust, on the order of micronewtons. It provides very fine attitude control or efficient acceleration of small spacecraft over long periods of time.

20 μN colloid thruster system.
[1]Flight use[edit]
Eight electrospray thrusters were first used in space on the NASA ST-7 ESA LISA Pathfinder mission, to demonstrate disturbance reduction.[2] Having logged roughly 1,400 hours on-orbit, the Busek built thrusters system met 100% of their mission goals for the LISA Pathfinder mission.[3]
By the end of April 2015, Busek had developed a smaller electrospray colloid thruster capable of generating 20 mN in a 17.8 x 17.8 x 4.3 cm (7"×7"×1.7") package.[4]
Colloid thrusters have also been commercialized by the company Accion Systems. Accion Systems' founder and CEO Natalya Bailey commercialized MIT Space Propulsion Laboratory technology to create the company's TILE (Tiled Ionic Liquid Electrospray) technology.
So far, Accion Systems has two thrusters in flight aboard CubeSats: Irvine01 and Irvine02. These two spacecraft are developed by a group of high schools from Irvine, California, as part of the Irvine CubeSat STEM Program.
Experiments[edit]
In July 2013, scientists from Michigan Technological University and the University of Maryland led by Kurt Terhune demonstrated an electrospray system within a transmission electron microscope (TEM). This led to the discovery that the TEM environment formed needle-like structures on the thruster disrupting the way the electrospray system works.[5]
The SkyFire nanosatellite, to be launched in 2021 for a lunar flyby, will demonstrate the use of this propulsion system.[6]
See also[edit]
https://en.wikipedia.org/wiki/Colloid_thruster
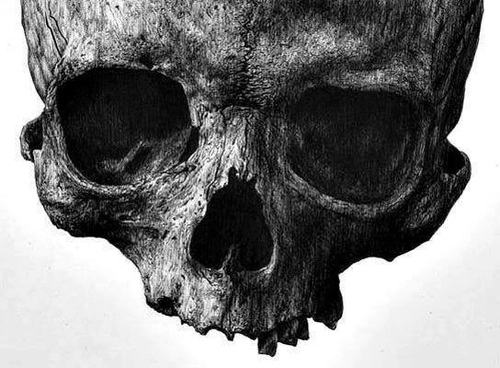
In mass spectrometry, matrix-assisted laser desorption/ionization (MALDI) is an ionization technique that uses a laser energy absorbing matrix to create ions from large molecules with minimal fragmentation.[1] It has been applied to the analysis of biomolecules (biopolymers such as DNA, proteins, peptides and carbohydrates) and various organic molecules (such as polymers, dendrimers and other macromolecules), which tend to be fragile and fragment when ionized by more conventional ionization methods. It is similar in character to electrospray ionization(ESI) in that both techniques are relatively soft (low fragmentation) ways of obtaining ions of large molecules in the gas phase, though MALDI typically produces far fewer multi-charged ions.
MALDI methodology is a three-step process. First, the sample is mixed with a suitable matrix material and applied to a metal plate. Second, a pulsed laser irradiates the sample, triggering ablation and desorption of the sample and matrix material. Finally, the analyte molecules are ionized by being protonated or deprotonated in the hot plume of ablated gases, and then they can be accelerated into whichever mass spectrometer is used to analyse them.[2]
https://en.wikipedia.org/wiki/Matrix-assisted_laser_desorption/ionization

Desorption is a phenomenon whereby a substance is released from or through a surface. The process is the opposite of sorption (that is, either adsorption or absorption). This occurs in a system being in the state of sorption equilibrium between bulk phase (fluid, i.e. gas or liquid solution) and an adsorbing surface (solid or boundary separating two fluids). When the concentration (or pressure) of substance in the bulk phase is lowered, some of the sorbed substance changes to the bulk state. The capability of desorbing is termed desorptive capacity.
In chemistry, especially chromatography, desorption is the ability for a chemical to move with the mobile phase. The more a chemical desorbs, the less likely it will adsorb, thus instead of sticking to the stationary phase, the chemical moves up with the solvent front.
In chemical separation processes, stripping is also referred to as desorption as one component of a liquid stream moves by mass transfer into a vapor phase through the liquid-vapor interface.
After adsorption, the adsorbed chemical will remain on the substrate nearly indefinitely, provided the temperature remains low. However, as the temperature rises, so does the likelihood of desorption. The general equation for the rate of desorption is:

where
is the rate constant for desorption,
is the concentration of the adsorbed material, and
is the kinetic order of desorption.
Usually, the order of the desorption can be predicted by the number of elementary steps involved:
Atomic or simple molecular desorption will typically be a first-order process (i.e., a simple molecule on the surface of the substrate desorbs into a gaseous form).
Recombinative molecular desorption will generally be a second-order process (i.e., two hydrogen atoms on the surface desorb and form a gaseous H2molecule).
The rate constant
may be expressed in the form

where
is the "attempt frequency" (often the Greek letter
), the chance of the adsorbed molecule overcoming its potential barrier to desorption,
is the activation energy of desorption,
is the Boltzmann constant, and
is the temperature.[1]
https://en.wikipedia.org/wiki/Desorption
https://en.wikipedia.org/wiki/Ablation
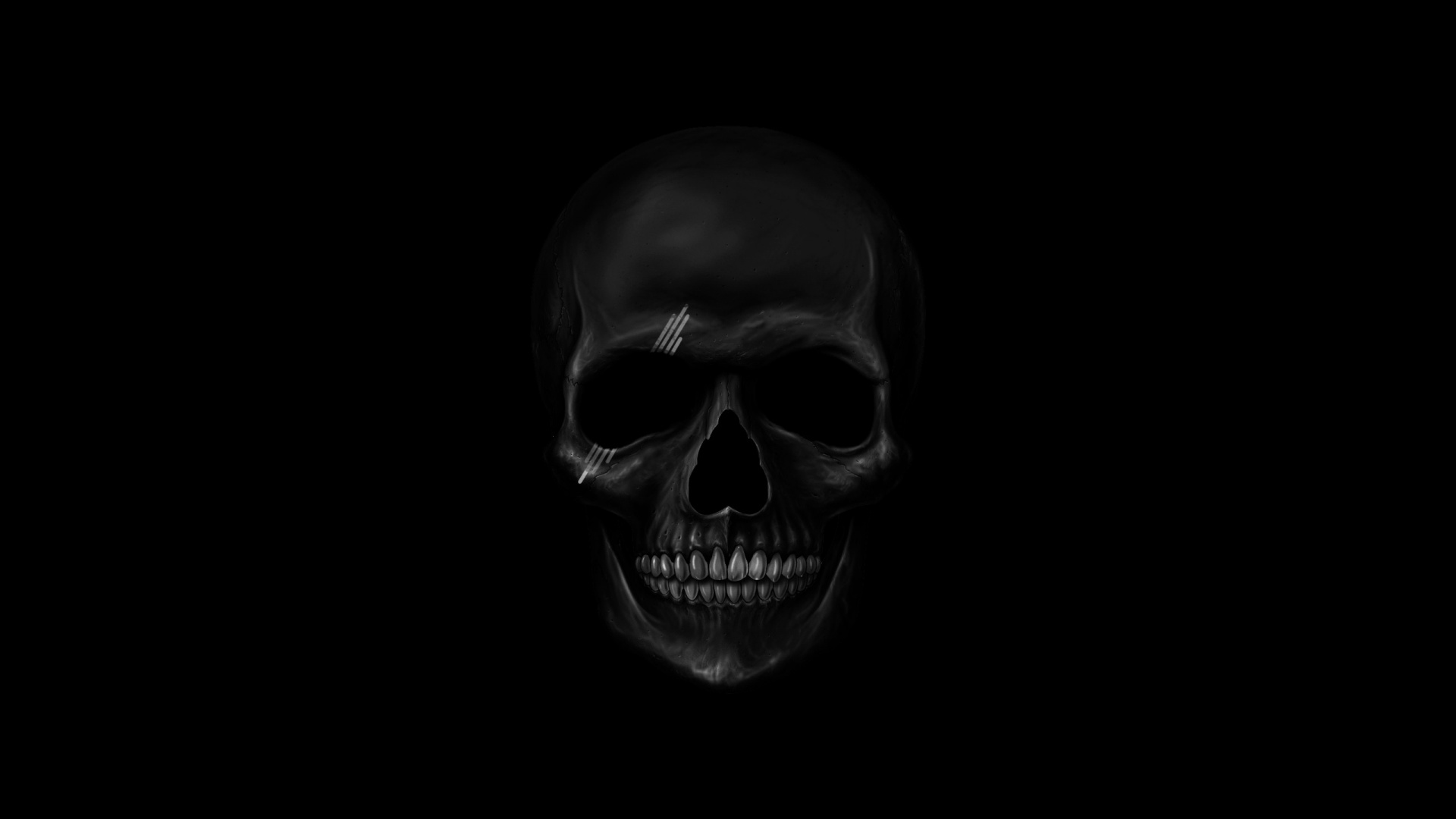
In chemistry, protonation (or hydronation) is the addition of a proton (or hydron, or hydrogen cation), (H+) to an atom, molecule, or ion, forming a conjugate acid.[1] (The complementary process, when a proton is removed from a Brønsted–Lowry acid, is deprotonation.) Some examples include
Protonation is a fundamental chemical reaction and is a step in many stoichiometric and catalytic processes. Some ions and molecules can undergo more than one protonation and are labeled polybasic, which is true of many biological macromolecules. Protonation and deprotonation (removal of a proton) occur in most acid–base reactions; they are the core of most acid–base reaction theories. A Brønsted–Lowry acid is defined as a chemical substance that protonates another substance. Upon protonating a substrate, the mass and the charge of the species each increase by one unit, making it an essential step in certain analytical procedures such as electrospray mass spectrometry. Protonating or deprotonating a molecule or ion can change many other chemical properties, not just the charge and mass, for example solubility, hydrophilicity, reduction potential, and optical properties can change.
https://en.wikipedia.org/wiki/Protonation
https://en.wikipedia.org/wiki/Deprotonation
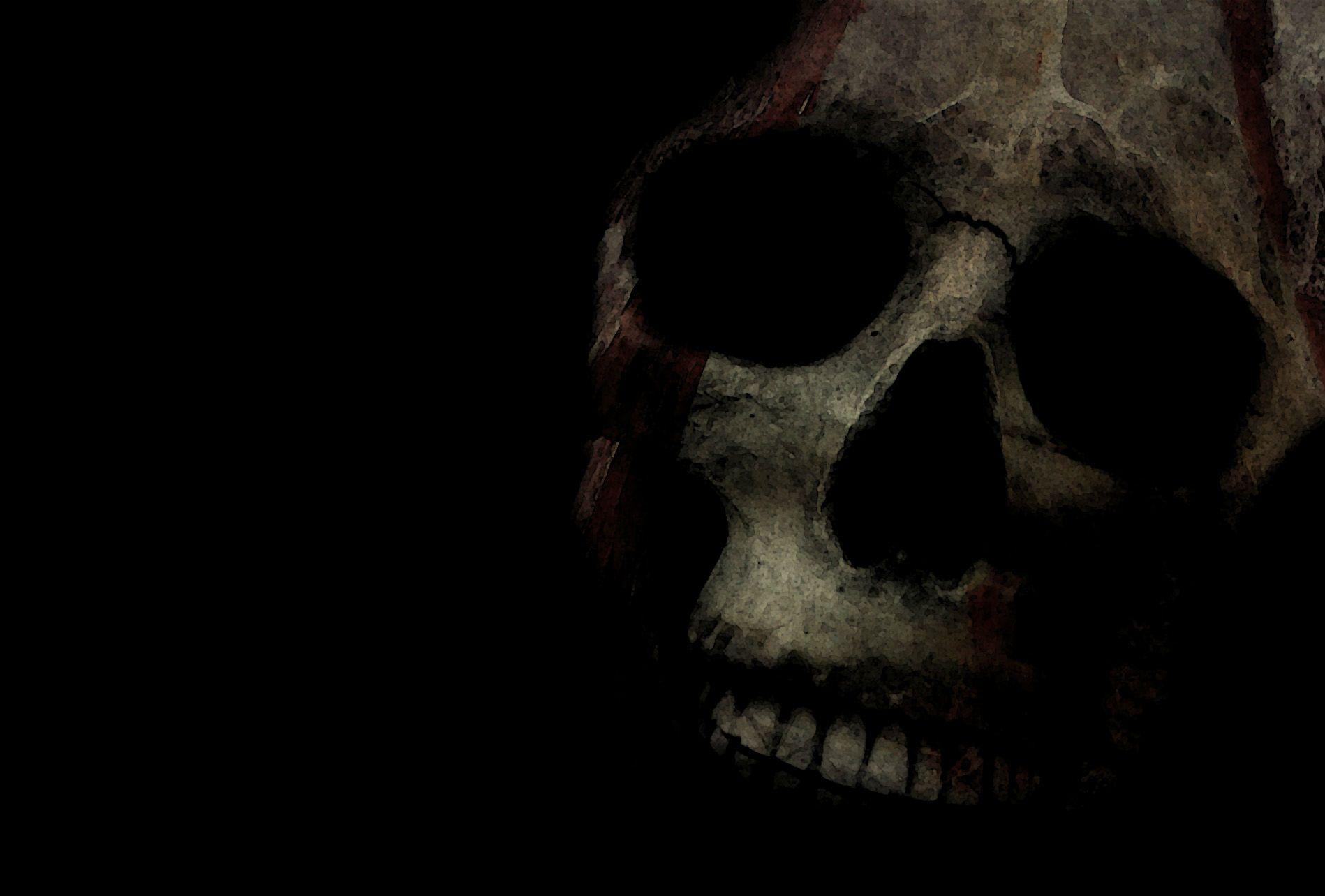
https://en.wikipedia.org/wiki/Matrix_(mass_spectrometry)
https://en.wikipedia.org/wiki/Crystal
https://en.wikipedia.org/wiki/Matrix-assisted_laser_desorption/ionization
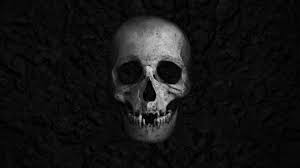
Dehydrogenation is the a chemical reaction that involves the removal of hydrogen, usually from an organic molecule. It is the reverse of hydrogenation. Dehydrogenation is important, both as a useful reaction and a serious problem. At its simplest, it is useful way of converting alkanes, which are relatively inert and thus low-valued, to olefins, which are reactive and thus more valuable. Alkenes are precursors to aldehydes, alcohols, polymers, and aromatics.[1] As a problematic reaction, the fouling and inactivation of many catalysts arises via coking, which is the dehydrogenative polymerization of organic substrates.[2]
Enzymes that catalyze dehydrogenation are called dehydrogenases.
Heterogeneous catalytic routes[edit]
Styrene[edit]
Dehydrogenation processes are used extensively to produce aromatics in the petrochemical industry. Such processes are highly endothermic and require temperatures of 500 °C and above.[1][3] Dehydrogenation also converts saturated fats to unsaturated fats. One of the largest scale dehydrogenation reactions is the production of styrene by dehydrogenation of ethylbenzene. Typical dehydrogenation catalysts are based on iron(III) oxide, promoted by several percent potassium oxide or potassium carbonate.[4]
- C6H5CH2CH3 → C6H5CH=CH2 + H2
Other alkenes[edit]
The importance of catalytic dehydrogenation of paraffin hydrocarbons to olefins has been growing steadily in recent years. Light olefins, such as butenes, are important raw materials for the synthesis of polymers, gasoline additives and various other petrochemical products. The cracking processes especially fluid catalytic cracking and steam cracker produce high-purity mono-olefins, such as 1-butene or isobutene. Despite such processes, currently more research is focused on developing alternatives such as oxidative dehydrogenation (ODH) for two reasons: (1) undesired reactions take place at high temperature leading to coking and catalyst deactivation, making frequent regeneration of the catalyst unavoidable, (2) it consumes a large amount of heat and requires high reaction temperatures. Oxidative dehydrogenation (ODH) of n-butane is an alternative to classical dehydrogenation, steam cracking and fluid catalytic cracking processes.[5] Propane[6]
Dehydrogenation of paraffins and olefins — paraffins such as n-pentane and isopentane can be converted to pentene and isopentene using chromium(III) oxide as a catalyst at 500 °C.
Formaldehyde[edit]
Formaldehyde is produced industrially by the catalytic oxidation of methanol, which can also be viewed as a dehydrogenation using O2 as the acceptor. The most common catalysts are silver metal or a mixture of an iron and molybdenum or vanadium oxides. In the commonly used formox process, methanol and oxygen react at ca. 250–400 °C in presence of iron oxide in combination with molybdenum and/or vanadium to produce formaldehyde according to the chemical equation:[7]
- 2 CH3OH + O2 → 2 CH2O + 2 H2O
Homogeneous catalytic routes[edit]
A variety of dehydrogenation processes have been described for organic compounds. These dehydrogenation is of interest in the synthesis of fine organic chemicals.[8] Such reactions often rely on transition metal catalysts.[9][10] Dehydrogenation of unfunctionalized alkanes can be effected by homogeneous catalysis. Especially active for this reaction are pincer complexes.[11][12]
Stoichiometric processes[edit]
Dehydrogenation of amines to nitriles using a variety of reagents, such as Iodine pentafluoride (IF
5).
In typical aromatization, six-membered alicyclic rings, e.g. cyclohexene, can be aromatized in the presence of hydrogenation acceptors. The elements sulfur and selenium promote this process. On the laboratory scale, quinones, especially 2,3-Dichloro-5,6-dicyano-1,4-benzoquinone (DDQ) are effective.

Main group hydrides[edit]
The dehydrogenative coupling of silanes has also been developed.[13]
- n PhSiH3 → [PhSiH]n + n H2
The dehydrogenation of amine-boranes is related reaction. This process once gained interests for its potential for hydrogen storage.[14]
https://en.wikipedia.org/wiki/Dehydrogenation
https://en.wikipedia.org/wiki/Category:Hydrogen
https://en.wikipedia.org/wiki/deuterium

Cold fusion is a hypothesized type of nuclear reaction that would occur at, or near, room temperature. It would contrast starkly with the "hot" fusion that is known to take place naturally within stars and artificially in hydrogen bombs and prototype fusion reactors under immense pressure and at temperatures of millions of degrees, and be distinguished from muon-catalyzed fusion. There is currently no accepted theoretical model that would allow cold fusion to occur.
History[edit]
Nuclear fusion is normally understood to occur at temperatures in the tens of millions of degrees. This is called "thermonuclear fusion". Since the 1920s, there has been speculation that nuclear fusion might be possible at much lower temperatures by catalytically fusing hydrogen absorbed in a metal catalyst. In 1989, a claim by Stanley Pons and Martin Fleischmann (then one of the world's leading electrochemists) that such cold fusion had been observed caused a brief media sensation before the majority of scientists criticized their claim as incorrect after many found they could not replicate the excess heat. Since the initial announcement, cold fusion research has continued by a small community of researchers who believe that such reactions happen and hope to gain wider recognition for their experimental evidence.
Early research[edit]
The ability of palladium to absorb hydrogen was recognized as early as the nineteenth century by Thomas Graham.[21] In the late 1920s, two Austrian-born scientists, Friedrich Paneth and Kurt Peters, originally reported the transformation of hydrogen into helium by nuclear catalysis when hydrogen was absorbed by finely divided palladium at room temperature. However, the authors later retracted that report, saying that the helium they measured was due to background from the air.
In 1927 Swedish scientist John Tandberg reported that he had fused hydrogen into helium in an electrolytic cell with palladium electrodes. On the basis of his work, he applied for a Swedish patent for "a method to produce helium and useful reaction energy". Due to Paneth and Peters's retraction and his inability to explain the physical process, his patent application was denied.[23] After deuterium was discovered in 1932, Tandberg continued his experiments with heavy water. The final experiments made by Tandberg with heavy water were similar to the original experiment by Fleischmann and Pons.[24] Fleischmann and Pons were not aware of Tandberg's work.[25][text 1][text 2]
The term "cold fusion" was used as early as 1956 in an article in The New York Times about Luis Alvarez's work on muon-catalyzed fusion. Paul Palmer and then Steven Jones of Brigham Young University used the term "cold fusion" in 1986 in an investigation of "geo-fusion", the possible existence of fusion involving hydrogen isotopes in a planetary core. In his original paper on this subject with Clinton Van Siclen, submitted in 1985, Jones had coined the term "piezonuclear fusion".[28]
https://en.wikipedia.org/wiki/Cold_fusion

Pyroelectric fusion refers to the technique of using pyroelectric crystals to generate high strength electrostatic fields to accelerate deuterium ions(tritium might also be used someday) into a metal hydride target also containing deuterium (or tritium) with sufficient kinetic energy to cause these ions to undergo nuclear fusion. It was reported in April 2005 by a team at UCLA. The scientists used a pyroelectric crystal heated from −34 to 7 °C (−29 to 45 °F), combined with a tungsten needle to produce an electric field of about 25 gigavolts per meter to ionize and accelerate deuterium nuclei into an erbium deuteride target. Though the energy of the deuterium ions generated by the crystal has not been directly measured, the authors used 100 keV (a temperature of about 109 K) as an estimate in their modeling.[1] At these energy levels, two deuterium nuclei can fuse together to produce a helium-3nucleus, a 2.45 MeV neutron and bremsstrahlung. Although it makes a useful neutron generator, the apparatus is not intended for power generation since it requires far more energy than it produces.[2][3][4][5]
https://en.wikipedia.org/wiki/Pyroelectric_fusion
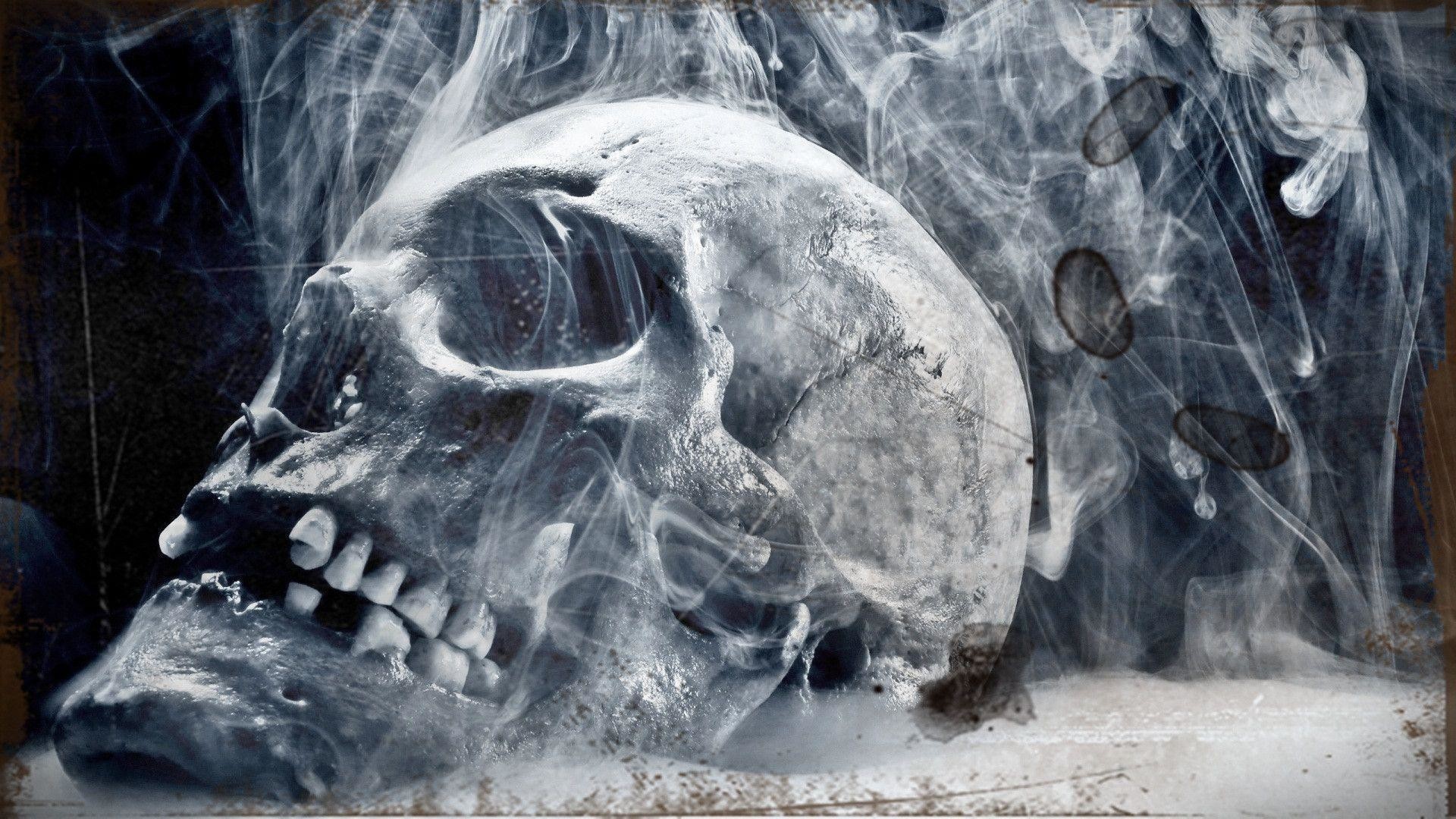
Muon-catalyzed fusion (abbreviated as μCF) is a process allowing nuclear fusion to take place at temperatures significantly lower than the temperatures required for thermonuclear fusion, even at room temperature or lower. It is one of the few known ways of catalyzing nuclear fusion reactions.
Muons are unstable subatomic particles which are similar to electrons but 207 times more massive. If a muon replaces one of the electrons in a hydrogen molecule, the nuclei are consequently drawn 196[1][2] times closer than in a normal molecule, due to the reduced mass being 196 times the mass of an electron. When the nuclei are this close together, the probability of nuclear fusion is greatly increased, to the point where a significant number of fusion events can happen at room temperature.
Current methods for obtaining muons, however, require far more energy than can be produced by the resulting catalyzed nuclear fusion reactions, and this is one of the main reasons muon catalyzed fusion reactors haven't been constructed. To create useful room-temperature muon-catalyzed fusion, reactors would need a cheaper, more efficient muon source and/or a way for each individual muon to catalyze many more fusion reactions. Laser-driven muon sources seem to be the economical tipping point for making muon-catalyzed fusion reactors viable.[citation needed]
https://en.wikipedia.org/wiki/Muon-catalyzed_fusion
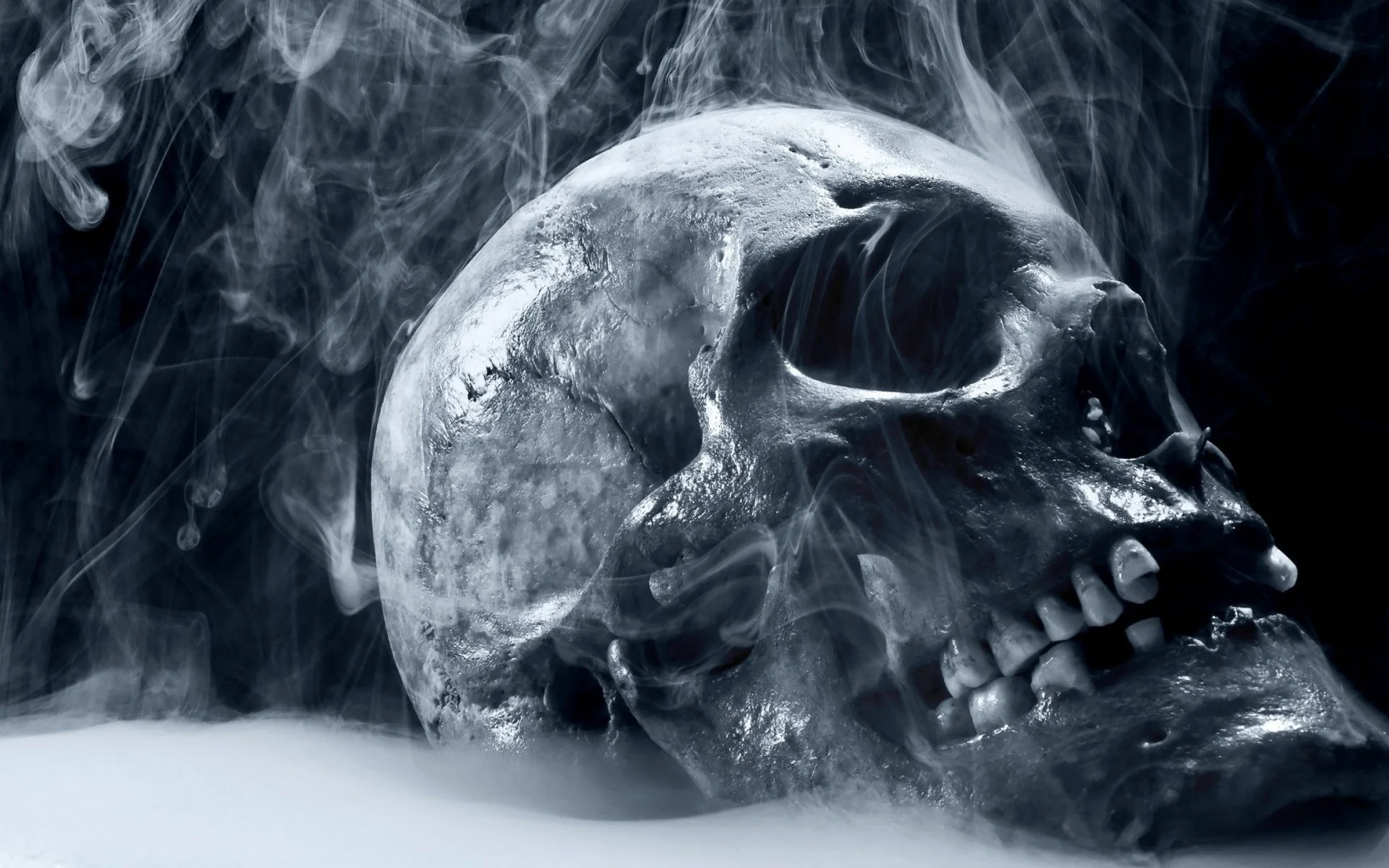
One practical problem with the muon-catalyzed fusion process is that muons are unstable, decaying in 2.2 μs (in their rest frame).[7] Hence, there needs to be some cheap means of producing muons, and the muons must be arranged to catalyze as many nuclear fusion reactions as possible before decaying.
Another, and in many ways more serious, problem is the "alpha-sticking" problem, which was recognized by Jackson in his 1957 paper.[6][note 3] The α-sticking problem is the approximately 1% probability of the muon "sticking" to the alpha particle that results from deuteron-triton nuclear fusion, thereby effectively removing the muon from the muon-catalysis process altogether. Even if muons were absolutely stable, each muon could catalyze, on average, only about 100 d-t fusions before sticking to an alpha particle, which is only about one-fifth the number of muon catalyzed d-t fusions needed for break-even, where as much thermal energy is generated as electrical energy is consumed to produce the muons in the first place, according to Jackson's rough estimate.[6]
More recent measurements seem to point to more encouraging values for the α-sticking probability, finding the α-sticking probability to be around 0.3% to 0.5%, which could mean as many as about 200 (even up to 350) muon-catalyzed d-t fusions per muon.[8] Indeed, the team led by Steven E. Jonesachieved 150 d-t fusions per muon (average) at the Los Alamos Meson Physics Facility.[9] The results were promising and almost enough to reach theoretical break-even. Unfortunately, these measurements for the number of muon-catalyzed d-t fusions per muon are still not enough to reach industrial break-even. Even with break-even, the conversion efficiency from thermal energy to electrical energy is only about 40% or so, further limiting viability. The best recent estimates of the electrical "energy cost" per muon is about 6 GeV with accelerators that are (coincidentally) about 40% efficient at transforming electrical energy from the power grid into acceleration of the deuterons.
As of 2012, no practical method of producing energy through this means has been published, although some discoveries using the Hall effect show promise.[10][failed verification]
Process[edit]
To create this effect, a stream of negative muons, most often created by decaying pions, is sent to a block that may be made up of all three hydrogen isotopes (protium, deuterium, and/or tritium), where the block is usually frozen, and the block may be at temperatures of about 3 kelvin (−270 degrees Celsius) or so. The muon may bump the electron from one of the hydrogen isotopes. The muon, 207 times more massive than the electron, effectively shields and reduces the electromagnetic repulsion between two nuclei and draws them much closer into a covalent bond than an electron can. Because the nuclei are so close, the strong nuclear force is able to kick in and bind both nuclei together. They fuse, release the catalytic muon (most of the time), and part of the original mass of both nuclei is released as energetic particles, as with any other type of nuclear fusion. The release of the catalytic muon is critical to continue the reactions. The majority of the muons continue to bond with other hydrogen isotopes and continue fusing nuclei together. However, not all of the muons are recycled: some bond with other debris emitted following the fusion of the nuclei (such as alpha particles and helions), removing the muons from the catalytic process. This gradually chokes off the reactions, as there are fewer and fewer muons with which the nuclei may bond. The number of reactions achieved in the lab can be as high as 150 d-t fusions per muon (average).
Deuterium-tritium (d-t or dt)[edit]
In the muon-catalyzed fusion of most interest, a positively charged deuteron (d), a positively charged triton (t), and a muon essentially form a positively charged muonic molecular heavy hydrogen ion (d-μ-t)+. The muon, with a rest mass 207 times greater than the rest mass of an electron,[7] is able to drag the more massive triton and deuteron 207 times closer together to each other[1] [2] in the muonic (d-μ-t)+ molecular ion than can an electron in the corresponding electronic (d-e-t)+ molecular ion. The average separation between the triton and the deuteron in the electronic molecular ion is about one angstrom (100 pm),[6][note 4] so the average separation between the triton and the deuteron in the muonic molecular ion is 207 times smaller than that.[note 5] Due to the strong nuclear force, whenever the triton and the deuteron in the muonic molecular ion happen to get even closer to each other during their periodic vibrational motions, the probability is very greatly enhanced that the positively charged triton and the positively charged deuteron would undergo quantum tunnelling through the repulsive Coulomb barrier that acts to keep them apart. Indeed, the quantum mechanical tunnelling probability depends roughly exponentially on the average separation between the triton and the deuteron, allowing a single muon to catalyze the d-t nuclear fusion in less than about half a picosecond, once the muonic molecular ion is formed.[6]
The formation time of the muonic molecular ion is one of the "rate-limiting steps" in muon-catalyzed fusion that can easily take up to ten thousand or more picoseconds in a liquid molecular deuterium and tritium mixture (D2, DT, T2), for example.[6] Each catalyzing muon thus spends most of its ephemeral existence of 2.2 microseconds,[7] as measured in its rest frame, wandering around looking for suitable deuterons and tritons with which to bind.
Another way of looking at muon-catalyzed fusion is to try to visualize the ground state orbit of a muon around either a deuteron or a triton. Suppose the muon happens to have fallen into an orbit around a deuteron initially, which it has about a 50% chance of doing if there are approximately equal numbers of deuterons and tritons present, forming an electrically neutral muonic deuterium atom (d-μ)0 that acts somewhat like a "fat, heavy neutron" due both to its relatively small size (again, 207 times smaller than an electrically neutral electronic deuterium atom (d-e)0) and to the very effective "shielding" by the muon of the positive charge of the proton in the deuteron. Even so, the muon still has a much greater chance of being transferred to any triton that comes near enough to the muonic deuterium than it does of forming a muonic molecular ion. The electrically neutral muonic tritium atom (t-μ)0 thus formed will act somewhat like an even "fatter, heavier neutron," but it will most likely hang on to its muon, eventually forming a muonic molecular ion, most likely due to the resonant formation of a hyperfine molecular state within an entire deuterium molecule D2 (d=e2=d), with the muonic molecular ion acting as a "fatter, heavier nucleus" of the "fatter, heavier" neutral "muonic/electronic" deuterium molecule ([d-μ-t]=e2=d), as predicted by Vesman, an Estonian graduate student, in 1967.[12]
Once the muonic molecular ion state is formed, the shielding by the muon of the positive charges of the proton of the triton and the proton of the deuteron from each other allows the triton and the deuteron to tunnel through the Coulomb barrier in time span of order of a nanosecond[13] The muon survives the d-t muon-catalyzed nuclear fusion reaction and remains available (usually) to catalyze further d-t muon-catalyzed nuclear fusions. Each exothermic d-t nuclear fusion releases about 17.6 MeV of energy in the form of a "very fast" neutron having a kinetic energy of about 14.1 MeV and an alpha particle α (a helium-4 nucleus) with a kinetic energy of about 3.5 MeV.[6] An additional 4.8 MeV can be gleaned by having the fast neutrons moderated in a suitable "blanket" surrounding the reaction chamber, with the blanket containing lithium-6, whose nuclei, known by some as "lithions," readily and exothermically absorb thermal neutrons, the lithium-6 being transmuted thereby into an alpha particle and a triton.[note 6]
Deuterium-deuterium and other types[edit]
The first kind of muon-catalyzed fusion to be observed experimentally, by L.W. Alvarez et al.,[5] was protium (H or 1H1) and deuterium (D or 1H2) muon-catalyzed fusion. The fusion rate for p-d (or pd) muon-catalyzed fusion has been estimated to be about a million times slower than the fusion rate for d-t muon-catalyzed fusion.[6][note 7]
Of more practical interest, deuterium-deuterium muon-catalyzed fusion has been frequently observed and extensively studied experimentally, in large part because deuterium already exists in relative abundance and, like hydrogen, deuterium is not at all radioactive. (Tritium rarely occurs naturally, and is radioactive with a half-life of about 12.5 years.[7])
The fusion rate for d-d muon-catalyzed fusion has been estimated to be only about 1% of the fusion rate for d-t muon-catalyzed fusion, but this still gives about one d-d nuclear fusion every 10 to 100 picoseconds or so.[6] However, the energy released with every d-d muon-catalyzed fusion reaction is only about 20% or so of the energy released with every d-t muon-catalyzed fusion reaction.[6] Moreover, the catalyzing muon has a probability of sticking to at least one of the d-d muon-catalyzed fusion reaction products that Jackson in this 1957 paper[6] estimated to be at least 10 times greater than the corresponding probability of the catalyzing muon sticking to at least one of the d-t muon-catalyzed fusion reaction products, thereby preventing the muon from catalyzing any more nuclear fusions. Effectively, this means that each muon catalyzing d-d muon-catalyzed fusion reactions in pure deuterium is only able to catalyze about one-tenth of the number of d-t muon-catalyzed fusion reactions that each muon is able to catalyze in a mixture of equal amounts of deuterium and tritium, and each d-d fusion only yields about one-fifth of the yield of each d-t fusion, thereby making the prospects for useful energy release from d-d muon-catalyzed fusion at least 50 times worse than the already dim prospects for useful energy release from d-t muon-catalyzed fusion.
Potential "aneutronic" (or substantially aneutronic) nuclear fusion possibilities, which result in essentially no neutrons among the nuclear fusion products, are almost certainly not very amenable to muon-catalyzed fusion.[6] One such essentially aneutronic nuclear fusion reaction involves a deuteron from deuterium fusing with a helion (h+2) from helium-3, which yields an energetic alpha particle and a much more energetic proton, both positively charged (with a few neutrons coming from inevitable d-d nuclear fusion side reactions). However, one muon with only one negative electric charge is incapable of shielding both positive charges of a helion from the one positive charge of a deuteron. The chances of the requisite two muons being present simultaneously are exceptionally remote.
- ^ The breeding takes place due to certain neutron-capture nuclear reactions, followed by beta decays, the ejection of electrons and neutrinos from nuclei as neutrons within the nuclei decay into protons as a result of weak nuclear forces.
- ^ Muons are not mesons; they are leptons. However, this was not clear until 1947, and the name "mu meson" was still used for some time following the identification of the muon as a lepton.
- ^ Eugene P. Wigner pointed out the α-sticking problem to Jackson.[citation needed]
- ^ According to Cohen, S.; Judd, D.L.; Riddell, Jr., R.J. (1960). "μ-Mesonic Molecules. II. Molecular-Ion Formation and Nuclear Catalysis". Phys. Rev. 119 (1): 397. Bibcode:1960PhRv..119..397C. doi:10.1103/PhysRev.119.397., footnote 16, Jackson may have been overly optimistic in Appendix D of his 1957 paper in his roughly calculated "guesstimate" of the rate of formation of a muonic (p-μ-p)+ molecular ion by a factor of about a million or so.)
- ^ In other words, the separation in the muonic case is about 500 femtometers[citation needed]
- ^ "Thermal neutrons" are neutrons that have been "moderated" by giving up most of their kinetic energy in collisions with the nuclei of the "moderating materials" or moderators, cooling down to "room temperature" and having a thermalized kinetic energy of about 0.025 eV, corresponding to an average "temperature" of about 300 kelvins or so.
- ^ In principle, of course, p-d nuclear fusion could be catalyzed by the electrons present in DO "heavy-ish" water molecules that naturally occur at the level of 0.0154% in ordinary water (H2O). However, because the proton and the deuteron would be more than 200 times farther apart in the case of the electronicHDO molecule than in the case of the muonic (p-μ-d)+ molecular ion, Jackson estimates that the rate of p-d "electron"-catalyzed fusion (eCF) is about 38 orders of magnitude (1038) slower than the rate of p-d muon-catalyzed fusion (μCF), which Jackson estimates to be about 106 per second, so p-d "electron"-catalyzed fusions (eCF) would be expected to occur at a rate of about 10−32 per second, meaning that one p-d "electron"-catalyzed fusion (eCF) might occur once every 1024 years or so.
https://en.wikipedia.org/wiki/Muon-catalyzed_fusion
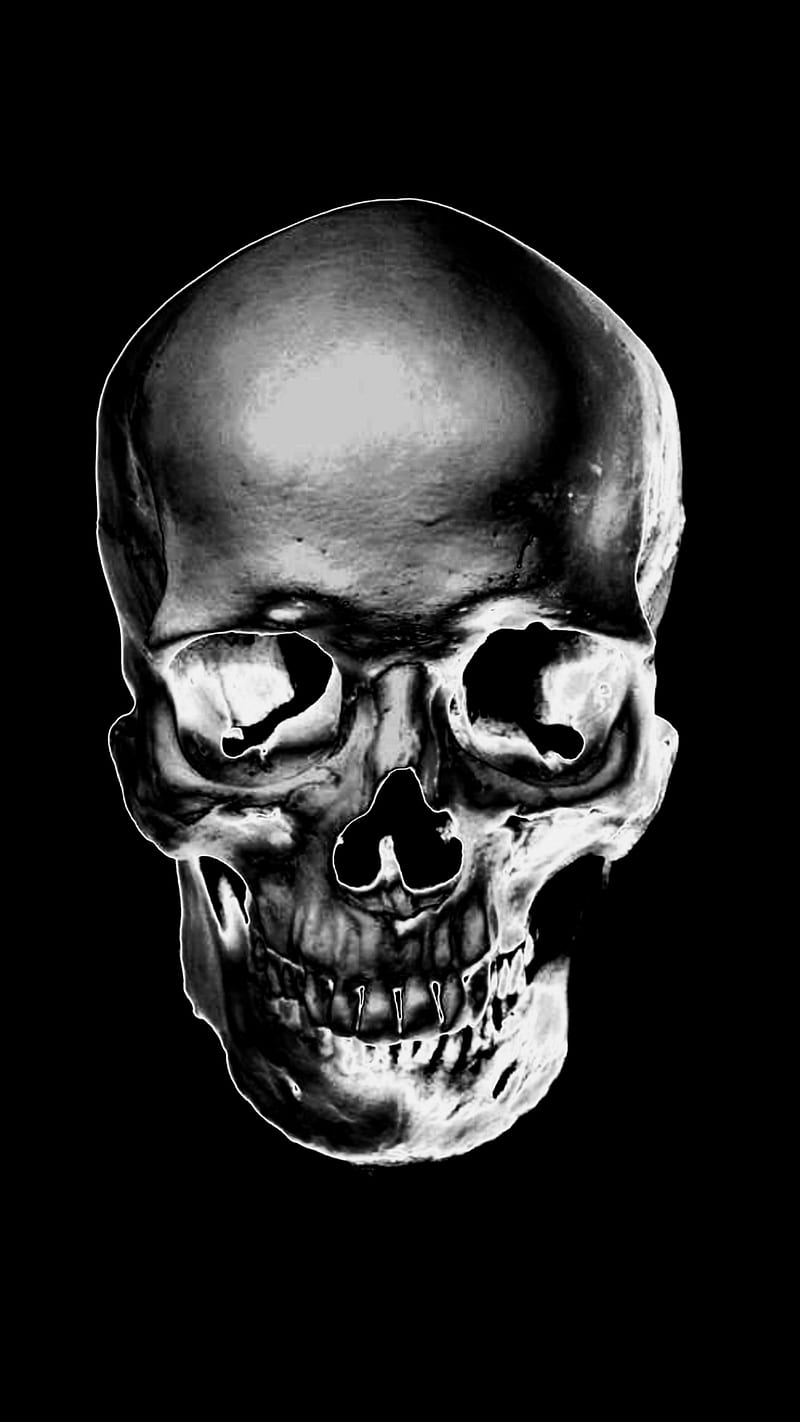
Fusion power, processes and devices
Core topics
Nuclear fusion Timeline List of experiments Nuclear power Nuclear reactor Atomic nucleus Fusion energy gain factor Lawson criterion Magnetohydrodynamics Neutron Plasma
Processes,
methods
Confinement
type
Gravitational
Alpha process Triple-alpha process CNO cycle Fusor Helium flash Nova remnants Proton-proton chain Carbon-burning Lithium burning Neon-burning Oxygen-burning Silicon-burning R-process S-process
Magnetic
Dense plasma focus Field-reversed configuration Levitated dipole Magnetic mirror Bumpy torus Reversed field pinch Spheromak Stellarator Tokamak Spherical Z-pinch
Inertial
Bubble (acoustic) Laser-driven Ion-driven Magnetized Liner Inertial Fusion
Electrostatic
Fusor Polywell
Other forms
Colliding beam Magnetized target Migma Muon-catalyzed Pyroelectric
https://en.wikipedia.org/wiki/Magnetohydrodynamics
https://en.wikipedia.org/wiki/Fusion_energy_gain_factor

Covid-19: France suspends 3,000 unvaccinated health workers
https://www.bbc.com/news/world-europe-58581682
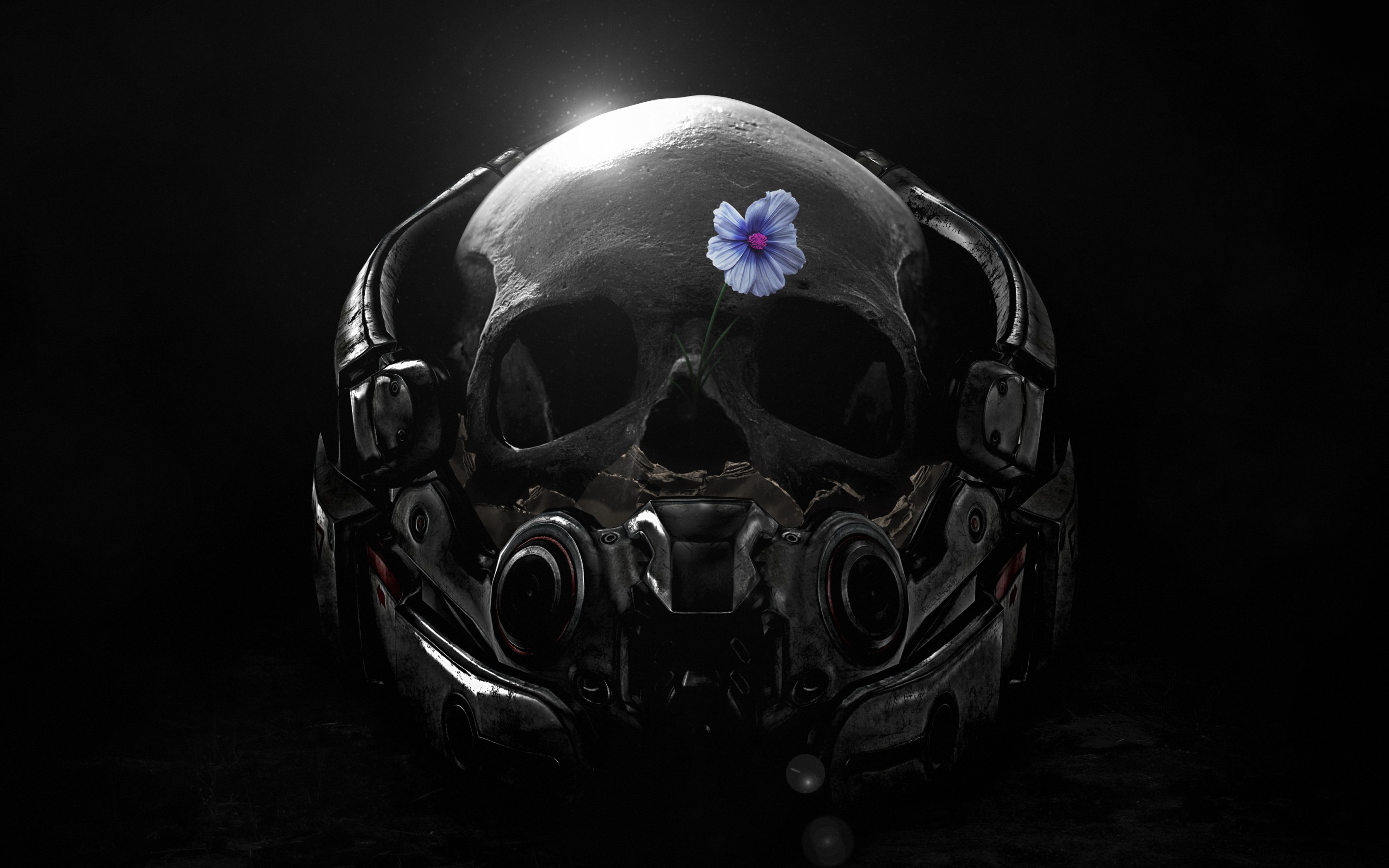
COVID-19: New Mu variant could be more vaccine-resistanthttps://news.un.org/en/story/2021/09/1098942
Nicki Minaj said Covid vaccine could make you impotent. Fauci shut her down.
"There’s no evidence that it happens, nor is there any mechanistic reason to imagine that it would happen," Dr. Anthony Fauci said.
https://www.nbcnews.com/news/us-news/nicki-minaj-said-covid-vaccine-could-make-you-impotent-fauci-n1279244
No comments:
Post a Comment