https://www.ecogate.com/post/what-is-the-fire-triangle
Fire Tirade - Oxyfenless Fires Alternate nucleo fule astrophy/whte drwarfs enth casc
The Nuclear Freeze campaign was a mass movement in the United States during the 1980s to planetearthy.
https://en.wikipedia.org/wiki/Nuclear_Freeze_campaign
Three processes are essential for the transfer of oxygen from the outside air to the blood flowing through the lungs: ventilation, diffusion, and perfusion.
Ventilation is the process by which air moves in and out of the lungs.
Diffusion is the spontaneous movement of gases, without the use of any energy or effort by the body, between the alveoli and the capillaries in the lungs.
Perfusion is the process by which the cardiovascular system pumps blood throughout the lungs.
https://www.merckmanuals.com/home/lung-and-airway-disorders/biology-of-the-lungs-and-airways/exchanging-oxygen-and-carbon-dioxide
https://wiki.oroboros.at/index.php/Oxygen_flux
DIW, Distance Inc W, Oxygen Triangles, Cascades, etc..
Arterial hypoxaemia in fibrotic lung disease is related more to ventilation-perfusion mismatching than to thickening of the alveolar wall.
Diffusion
Pulmonary oedema
Acute respiratory distress syndrome (particularly with fibrosis in later stages)
Ventilation-perfusion mismatch
Alveolar collapse
Acute respiratory distress syndrome
Pneumothorax
Obstructive airways disease
Drugs—pulmonary vasodilators
https://www.ncbi.nlm.nih.gov/pmc/articles/PMC1114207/
https://link.springer.com/chapter/10.1007/978-3-642-40717-8_2
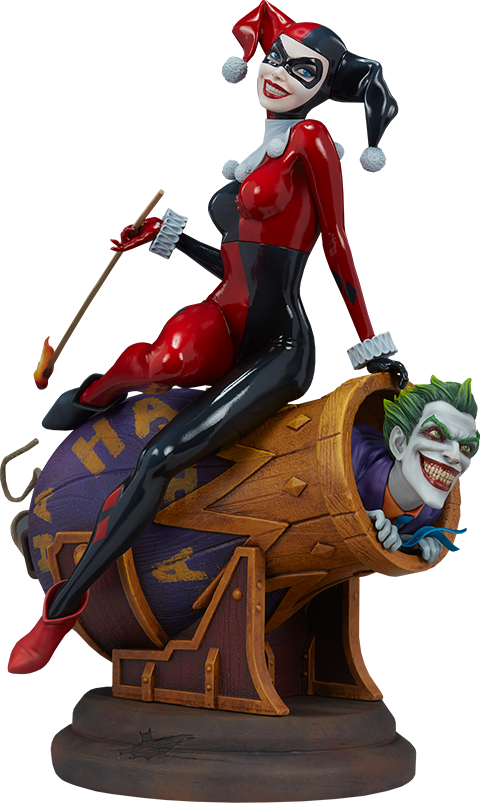

CSaturday, August 28 (2021); Communication and Ethics
"Critical incident defriefing" is the term used to describe a formal method of managing people who have been exposed to some sort of traumatic situation. In the history of the CICM Part II Exam, the trainees have been put in that position twice - in Question 28 from the second paper of 2014 and the identical Question 18 from the second paper of 2010. In these questions the candidates were asked to conduct a discussion with a junior trainee who had killed (or almost killed) somebody.
Apart from being somebody's creative outlet, Deranged Physiology is a place where one can find concentrated information about applied physiology which is for some reason difficult to track down in any other source. It is material which has been collected in the process of self-directed learning about the basic principles behind intensive care medicine, specifically the quintessential ICU stuff like mechanical ventilation, dialysis, inotropes, hemodynamic monitoring, et cetera et cetera. All too frequently this material exists in textbooks which for many are inaccessible, or in journal articles hidden behind paywalls. Occasionally the material is scattered across multiple sources.
Frequently, I forget where I got my information from. Because these notes started out as my own set of study materials for med school, without any intention of their being distributed on the internet, I had never referenced them accurately. Even now I am still very bad at it. If you read something I've published on this site and you discover that it is an unfair abuse of copyright, please write to me so I can make the necessary changes. What I don't want is intellectual property lawyers from the Oxford University Press kicking down my door one foggy morning.
https://derangedphysiology.com/main/recent
Polypropylene glycol or polypropylene oxide is the polymer of propylene glycol. Chemically it is a polyether, and, more generally speaking, it's a polyalkylene glycol (PAG) H S Code 3907.2000. The term polypropylene glycol or PPG is reserved for low to medium range molar mass polymer when the nature of the end-group, which is usually a hydroxyl group, still matters. The term "oxide" is used for high molar mass polymer when end-groups no longer affect polymer properties. Between 60 and 70% of propylene oxide is converted to polyether polyols by the process called alkoxylation.[1]
https://en.wikipedia.org/wiki/Polypropylene_glycol
Dr.Robert Bettey
Dr. Bettey, a native of Orange County, majored in mechanical engineering at Cal State Fullerton before receiving his Doctor of Veterinary Medicine Degree from UC Davis. He founded Equine Medical Associates after graduating and has been practicing in Orange County for over 25 years. His primary area of interest is lameness including advanced therapies for tendon injuries. He is one of only a few veterinarians in the country to have completed a post graduate Fellowship in equine ultrasound at the UC Davis School of Veterinary Medicine. Outside of work he enjoys family time, volleyball, and classic cars.
The first reference to this thing (the first time it is mentioned as a "cascade") is in Fenn & Rahn's Handbook of Physiology (vol. 2, 1965), where Luft used it to illustrate a chapter on aviation physiology.
...the best free peer-reviewed reference for this is probably the paper by George Biro (2013), the title of which ("From the atmosphere to the mitochondrion: the oxygen cascade") seemed to make it an ideal compass for this directionless chapter.
...Ortiz-Prado et al (2019) and official textbooks such as Chambers' Basic Physiology for Anaesthetists.
sea-level oxygen cascade events, which implies a starting atmospheric pressure of 760 mmHg. Without going off on a tangent, it will suffice to say that atmospheric oxygen content is 21%, which at sea level is (760 × 0.21) = 159.6 mmHg. This is the oxygen tension of dry room air, and represents the first stop on our "ordered journey".
If one were prone to pointless digressions, one could also illuminate this topic with a diagram of atmospheric oxygen tension plotted over increasing altitude, similar to the one from Ortiz-Prado et al (2019) but not copied directly from them because anybody can generate a graph from a spreadsheet:
Anatomical dead space gas; dilution by water vapour
The next stop on the journey is the decrease in the partial pressure of oxygen (as well as all the other gases, let's not forget them) by water vapour, which occurs in the course of the normal humidifier function of the upper airways. Though the oxygen cascade is usually represented as a series of steps, in actual fact there is a gradual slope of decreasing oxygen tension though the airways, down to the isothermic boundary where the partial pressure of water vapour is maximal. Borrowing data from Table 1 in Plotnikow et al (2018), one is again able to produce a chart of changing gas pressures on the way through the airway. It is somewhat inaccurate, given that
above. Nicki Minaj Barbie Tingz
The graph uses a relative humidity of 100% through most of the airway here, which is not entirely accurate, but is close enough for government work. In short, the decrease in oxygen content is relatively modest. The added 47 mmHg of water vapour pressure in the gas mixture decreases the pressure of all the other gases proportionally, and so the oxygen tension only decreases from 159 mmHg to 149 mmHg.
Alveolar gas; dilution by CO2 and absorption of O2
Logically, after the conducting airways the next step in the odyssey of oxygen is the alveolus, where the partial pressure of oxygen drops because of gas dilution by carbon dioxide. The partial pressure of carbon dioxide in the alveolus will obviously be quite variable, but under normal conditions the partial pressure of the alveolar oxygen will drop by about 50mmHg, down to 99. The precise extent of the drop can be established from the alveolar gas equation (Fehn, Rahn, and Otis, 1946). The modern form of the equation is as follows:
Thus, with 21% FiO2 and optimal
PAO2 = (0.21 x (760 - 47)) - (PaCO2 x 1.25)
Which ends up being
PAO2 = (149 - (PaCO2 x 1.25)
Thus, the patient with a relatively normal PaCO2 (say, 40 mmHg):
PAO2 = (149 - 50)
In short, the partial pressure of alveolar oxygen in this scenario is about 99 mmHg. Obviously, this is not going to be the case for every alveolus, as the alveoli which have high V/Q ratios (or those belonging to the alveolar dead space volume) cannot be expected to donate so much CO2 (or any of it) to the gas mixture. It is strange that, given how most of the time the same idealised parameters are chosen (760 mmHg atmospheric pressure, a PaCO2 of 40 mmHg), the textbooks all have a slightly different value for this step in the cascade. In fact, Nunn's, Levitzky and Wests' offer no specific oxygen cascade values for this step, Chambers give 99.7 mmHg, Brandis offers 100 mmHg and Part One have 105 mmHg.
In addition to CO2 moving into the alveolus, we must remember that oxygen is also removed from the alveolar gas. Apart from moving along a concentration gradient (from alveolar gas to the PaO2 of mixed venous blood, say 50 mmHg) there is also the uptake of oxygen by haemoglobin, which accounts for the majority of this drop. The magnitude of this drop is difficult to estimate for any given lung unit because of the many variables involved. All that can be safely said is that blood entering the lung has an oxygen content of around 97 ml/L (given a Hb of 100), and blood exiting the alveolar capillaries (probably) has an oxygen content of 130ml/L, with the extra oxygen coming from the alveolar gas. Given that there is 0.04464 mmol of oxygen in every 1ml, those 33 ml of oxygen (1.47 mmol) would be expected to exert a partial pressure of about 1.1 mmHg if the total gas mixture had a pressure of 760 mmHg.
Pulmonary capillary blood; diffusion gradient
Oxygen usually has little difficulty crossing the alveolar-capillary barrier, and therefore the difference between alveolar oxygen and endcapillary oxygen is usually minimal. So much so in fact that in most textbooks this step is omitted, and the shunt equation even assumes that it doesn't exist. That's probably quite a fair position to take, because by the end of the capillary the blood has had enough time (fractions of a second are required) to become maximally oxygenated.
To be sure, in everybody there is an alveolar-arterial PO2 difference, but it is due to other factors. Cohen et al (1971) explored this in healthy young men. The investigators measured the gross alveolar-arterial difference (about 9%) and then excluded the contribution of V/Q scatter and shunt by integrating test results taken with 100% FiO2. As one might recall, using an FiO2 of 100% essentially eliminates the contribution of V/Q scatter to the venous admixture, leaving behind only "true" shunt and things like alveolar-capillary diffusion. Cohen et al discovered that with 100% FiO2 these healthy young men had an A-a difference of 0 mmHg, i.e. their entire 9% difference was all due to V/Q scatter and there was no shunt and no diffusion problem whatsoever.
Only with exercise (which increases cardiac output and therefore decreases capillary transit time) did diffusion become an issue. The same young subjects were exposed to some "100 watt exercise" (defined as "bicycling, stationary, light effort" by Wikipedia), during which the authors were able to attribute an A-a difference of about 11 mmHg to diffusion.
Systemic arterial blood; venous admixture
After the diffusion step, the next step in the oxygen cascade should probably be the one produced by venous admixture. Under normal circumstances, venous admixturecontributes approximately 3% to the difference between alveolar and arterial PO2. This obviously changes in states of disease. Here, a lightly altered diagram stolen directly from Said & Banerjee (1963) demonstrates measured A-a differences in healthy patients as well as patients with broken lungs:
So, if one were using this diagram as a guide, one might be able to say that the systemic arterial PaO2 is 7 mmHg lower than the alveolar oxygen concentration (i.e around 92 mmHg) and that the majority of this difference was probably due to venous admixture from lung units with a V/Q ratio of less than 1.0. Beyond this step, arterial blood rapidly makes its way to the tissues, and it remains essentially unchanged on the way there.
The arterial-alveolar gradient (A-a gradient) should probably be mentioned here, even though it is discussed in greater detail elsewhere. In short, this is the gradient between measured arterial PO2 and the calculated alveolar PO2, determined through the use of the alveolar gas equation. Many generations of intensivists have grown up being forced to calculate this pointless metric, and a CICM trainee can expect for this trauma to be vertically trasmitted for many generations to come. All that needs to be said here is that in the college comments to Question 17 from the first paper of 2015 the examiners expected some mention of the A-a gradient, and its normal values. Without digressing extensively about the numerous possible age-related formulae, one can safely say that average value at 21% FiO2 is 7mmHg in the young, and 14mmHg in the old (Kanber et al, 1968).
Cells; diffusion gradient
Between the arterial blood and the cytoplasm of cells, there is a massive gradient of oxygen content, which drives the diffusion of oxygen into those tissues. An exact number is impossible to give, because all organs and tissues will be different, within each organ there will be regional variation, and even within the same region each cell will be slightly different in its distance from the nearest capillary (which is actually a major determinant). Thus, whatever partial pressure value one chooses to represent this step in the cascade, it will be wrong.
Being unable to recommend any one specific value to use for one's exam answer here, instead the author chose to confuse their reader with an overwhelming excess of values:
Organ/tissue | PaO2 (mmHg) |
Brain | 33.8 ± 2.6 |
Lung | 42.8 |
Skin (sub‐papillary plexus) | 35.2 ± 8 |
Skin (dermal papillae) | 24 ± 6.4 |
Skin (superficial region) | 8 ± 3.2 |
Intestinal tissue | 57.6 ± 2.3 |
Liver | 40.6 ± 5.4 |
Kidney | 72 ± 20 |
Muscle | 29.2 ± 1.8 |
Bone marrow | 48.9 ± 4.5 |
This list is from an excellent article by Carreau et al (2018), which has references to support each of the listed values.
Mitochondria; diffusion gradient
The last stop for oxygen is the mitochondria. Obviously, as tissues are different, so cells and mitochondria will be different, and beyond that measuring the actual mitochondrial oxygen concentration is tricky from a practical perspective. Dmitriev & Papkovsky (2012) reviewed some of the difficulties and found that a range of 1-10 mmHg is usually reported in the literature. Generally, textbooks tend to quote low numbers, for example Brandis gives 4-22 mmHg.
Critical oxygen deficit: "Pasteur point"
In this race to the bottom, how low can you go? Obviously, if you are experiencing cardiac arrest, it is not inconceivable that some of your mitochondria may be down to a PaO2 of 0 mmHg. Obviously, not all is well in those organelles, as aerobic metabolism must cease in the absence of substrate. But how low can oxygen tension get before the mitochondria stop trying to use it?
Most authors who go this far seem to describe some sort of a "Pasteur point", a critical threshold for oxygen tension beyond which aerobic metabolism cannot continue. This threshold appears to be governed by the affinity of the oxidative phosphorylation enzymes for oxygen, which is very high. Most people echo Brandis and give 1 mmHg as the lowest partial pressure of oxygen at which aerobic metabolism can continue.
The term "Pasteur point" is adapted from microbiology, and refers to the oxygen tension which is required for facultative anaerobic organisms to switch their metabolism from anaerobic fermentation to aerobic metabolism. The latter then suppresses the former, a phenomenon which has come to be known as the Pasteur effect (Engelhardt, 1974). Below this oxygen threshold, aerobic metabolism is viewed by these microorganisms as an unviable means of energy production, and is suspended. The 1mmHg partial pressure is also thought to be the critical point at which the oxygen enrichment of the Earth's atmosphere became sufficient to support complex life, at some stage in the deep Pre-Cambrian, perhaps around 600 million years ago (Rhoads & Morse, 1971). It would appear that modern microorganisms also have this built-in threshold, and by extension so do their distant relatives, the mitochondria. For mammalian mitochondria, this limit is probably around 7μmol/L O2, or 0.5 mmHg at normal body temperature. Wilson et al (1988) found that this was the concentration of oxygen required to suppress the respiratory activity of the cytochrome enzymes to half of the maximum.

A free online resource for Intensive Care Medicine
© Alex Yartsev
2013-2021
https://derangedphysiology.com/main/cicm-primary-exam/required-reading/respiratory-system/Chapter%20101/oxygen-cascade
https://www.nytimes.com/2016/08/07/movies/harley-quinn-suicide-squad.html
Fraction of inspired oxygen (FIO2), corrected denoted with a capital "I",[1] is the molar or volumetric fraction of oxygen in the inhaled gas. Medical patients experiencing difficulty breathing are provided with oxygen-enriched air, which means a higher-than-atmospheric FIO2. Natural air includes 21% oxygen, which is equivalent to FIO2 of 0.21. Oxygen-enriched air has a higher FIO2than 0.21; up to 1.00 which means 100% oxygen. FIO2 is typically maintained below 0.5 even with mechanical ventilation, to avoid oxygen toxicity,[2] but there are applications when up to 100% is routinely used.
Often used in medicine, the FIO2 is used to represent the percentage of oxygen participating in gas-exchange. If the barometric pressure changes, the FIO2 may remain constant while the partial pressure of oxygen changes with the change in barometric pressure.
https://en.wikipedia.org/wiki/Fraction_of_inspired_oxygen
Cold shock response is a series of cardio-respiratory responses caused by sudden immersion in cold water.
In cold water immersions, cold shock response is perhaps the most common cause of death,[1] such as by falling through thin ice. The immediate shock of the cold causes involuntary inhalation, which, if underwater, can result in drowning. The cold water can also cause heart attack due to vasoconstriction;[2] the heart has to work harder to pump the same volume of blood throughout the body. For people with existing cardiovascular disease, the additional workload can result in cardiac arrest. Inhalation of water (and thus drowning) may result from hyperventilation. Some people are much better able to survive swimming in very cold water due to body or mental conditioning.[1]
https://en.wikipedia.org/wiki/Cold_shock_response
The science of underwater diving includes those concepts which are useful for understanding the underwater environment in which diving takes place, and its influence on the diver. It includes aspects of physics, physiology and oceanography. The practice of scientific work while diving is known as Scientific diving. These topics are covered to a greater or lesser extent in diver trainingprograms, on the principle that understanding the concepts may allow the diver to avoid problems and deal with them more effectively when they cannot be avoided.
A basic understanding of the physics of the underwater environment is foundational to the understanding of the short and long term physiological effects on the diver, and the associated hazards of the diving environment and their consequences which are inherent to diving.
https://en.wikipedia.org/wiki/Science_of_underwater_diving
In physiology, isobaric counterdiffusion (ICD) is the diffusion of different gases into and out of tissues while under a constant ambient pressure, after a change of gas composition, and the physiological effects of this phenomenon. The term inert gas counterdiffusion is sometimes used as a synonym, but can also be applied to situations where the ambient pressure changes.[1][2] It has relevance in mixed gas diving and anesthesiology.
https://en.wikipedia.org/wiki/Isobaric_counterdiffusion
Diving medicine
Diving
disorders
List of signs and symptoms of diving disorders Cramp Motion sickness Surfer's ear
Pressure
related
Alternobaric vertigo Barostriction Barotrauma Air embolism Aerosinusitis Barodontalgia Dental barotrauma Pulmonary barotrauma Compression arthralgia Decompression illness Dysbarism
Oxygen
Freediving blackout Hyperoxia Hypoxia Oxygen toxicity
Inert gases
Avascular necrosis Decompression sickness Isobaric counterdiffusion Taravana Dysbaric osteonecrosis High-pressure nervous syndrome Hydrogen narcosis Nitrogen narcosis
Carbon dioxide
Hypercapnia Hypocapnia
Breathing gas
contaminants
Carbon monoxide poisoning
Immersion
related
Asphyxia Drowning Hypothermia Immersion diuresis Instinctive drowning response Laryngospasm Salt water aspiration syndrome Swimming-induced pulmonary edema
Treatment
Demand valve oxygen therapy First aid Hyperbaric medicine Hyperbaric treatment schedules In-water recompression Oxygen therapy Therapeutic recompression
Personnel
Diving Medical Examiner Diving Medical Practitioner Diving Medical Technician Hyperbaric nursing
Screening
Atrial septal defect Effects of drugs on fitness to dive Fitness to dive Psychological fitness to dive
Research
Researchers in
diving physiology
and medicine
Arthur J. Bachrach Albert R. Behnke Paul Bert George F. Bond Robert Boyle Albert A. Bühlmann John R. Clarke Guybon Chesney Castell Damant Kenneth William Donald William Paul Fife John Scott Haldane Robert William Hamilton Jr. Leonard Erskine Hill Brian Andrew Hills Felix Hoppe-Seyler Christian J. Lambertsen Simon Mitchell Charles Momsen John Rawlins R.N. Charles Wesley Shilling Edward D. Thalmann Jacques Triger
Diving medical
research
organisations
Aerospace Medical Association Divers Alert Network (DAN) Diving Diseases Research Centre (DDRC) Diving Medical Advisory Council (DMAC) European Diving Technology Committee (EDTC) European Underwater and Baromedical Society (EUBS) National Board of Diving and Hyperbaric Medical Technology Naval Submarine Medical Research Laboratory Royal Australian Navy School of Underwater Medicine Rubicon Foundation South Pacific Underwater Medicine Society (SPUMS) Southern African Underwater and Hyperbaric Medical Association (SAUHMA) Undersea and Hyperbaric Medical Society (UHMS) United States Navy Experimental Diving Unit (NEDU)
https://en.wikipedia.org/wiki/Fraction_of_inspired_oxygen
Salt water aspiration syndrome is a rare diving disorder suffered by scuba divers who inhale a mist of seawater from a faulty demand valve causing irritation of the lungs. It is not the same thing as aspiration of salt water as a bulk liquid, i.e. drowning.[1][2] It can be treated by rest for several hours. If severe, medical assessment is required.
https://en.wikipedia.org/wiki/Salt_water_aspiration_syndrome
High-pressure nervous syndrome (HPNS – also known as high-pressure neurological syndrome) is a neurological and physiological diving disorder which can result when a diver descends below about 500 feet (150 m) using a breathing gas containing helium. The effects experienced, and the severity of those effects, depend on the rate of descent, the depth and the percentage of helium.[1]
"Helium tremors" were first widely described in 1965 by Royal Navy physiologist Peter B. Bennett, who also founded the Divers Alert Network.[1][2] Russian scientist G. L. Zal'tsman also reported on helium tremors in his experiments from 1961. However, these reports were not available in the West until 1967.[3]
The term high-pressure nervous syndrome was first used by Brauer in 1968 to describe the combined symptoms of tremor, electroencephalography (EEG) changes, and somnolence that appeared during a 1,189-foot (362 m) chamber dive in Marseille.[4]
HPNS has two components, one resulting from the speed of compression and the other from the absolute pressure.
Those suggest that helium might cause substantial lipid membrane distortion. The high hydrostatic pressure itself has a less damaging influence on the membrane, reducing molecular volumes, but leaving the molecular boundary intact.[6]
https://en.wikipedia.org/wiki/High-pressure_nervous_syndrome
Hydrogen narcosis (also known as the hydrogen effect) is the psychotropic state induced by breathing hydrogen at high pressures. Hydrogen narcosis produces symptoms such as hallucinations, disorientation, and confusion, which are similar to hallucinogenic drugs. It can be experienced by deep-sea divers who dive to 300 m (1,000 ft) below sea level breathing hydrogen mixtures.[1] However, hydrogen has far less narcotic effect than nitrogen (which causes the better known nitrogen narcosis) and is very rarely used in diving. In tests of the effect of hydrogen narcosis, where divers dived to 500 m (1,600 ft) with a hydrogen–helium–oxygen (Hydreliox) mixture containing 49% hydrogen, it was found that while the narcotic effect of hydrogen was detectable, the neurological symptoms of high-pressure nervous syndrome were only moderate.[2][3]
https://en.wikipedia.org/wiki/Hydrogen_narcosis
Narcosis while diving (also known as nitrogen narcosis, inert gas narcosis, raptures of the deep, Martini effect) is a reversible alteration in consciousness that occurs while diving at depth. It is caused by the anesthetic effect of certain gases at high pressure. The Greek word νάρκωσις (narkōsis), "the act of making numb", is derived from νάρκη (narkē), "numbness, torpor", a term used by Homer and Hippocrates.[1] Narcosis produces a state similar to drunkenness (alcohol intoxication), or nitrous oxide inhalation. It can occur during shallow dives, but does not usually become noticeable at depths less than 30 meters (100 ft).
Except for helium and probably neon, all gases that can be breathed have a narcotic effect, although widely varying in degree.[2][3] The effect is consistently greater for gases with a higher lipid solubility, and although the mechanism of this phenomenon is still not fully clear, there is good evidence that the two properties are mechanistically related.[2] As depth increases, the mental impairment may become hazardous. Divers can learn to cope with some of the effects of narcosis, but it is impossible to develop a tolerance. Narcosis affects all divers, although susceptibility varies widely among individuals and from dive to dive.
Narcosis may be completely reversed in a few minutes by ascending to a shallower depth, with no long-term effects. Thus narcosis while diving in open water rarely develops into a serious problem as long as the divers are aware of its symptoms, and are able to ascend to manage it. Diving much beyond 40 m (130 ft) is generally considered outside the scope of recreational diving. In order to dive at greater depths, as narcosis and oxygen toxicity become critical risk factors, specialist training is required in the use of various helium-containing gas mixtures such as trimix or heliox. These mixtures prevent narcosis by replacing some or all of the inert fraction of the breathing gas with non-narcotic helium.
Narcosis results from breathing gases under elevated pressure, and may be classified by the principal gas involved. The noble gases, except helium and probably neon,[2] as well as nitrogen, oxygen and hydrogen cause a decrement in mental function, but their effect on psychomotor function (processes affecting the coordination of sensory or cognitive processes and motor activity) varies widely. The effect of carbon dioxide is a consistent diminution of mental and psychomotor function.[4] The noble gases argon, krypton, and xenon are more narcotic than nitrogen at a given pressure, and xenon has so much anesthetic activity that it is a usable anesthetic at 80% concentration and normal atmospheric pressure. Xenon has historically been too expensive to be used very much in practice, but it has been successfully used for surgical operations, and xenon anesthesia systems are still being proposed and designed.[5]
Some components of breathing gases and their relative narcotic potencies:[2][FN 1][3] | |
Gas | Relative narcotic potency |
---|---|
He | 0.045 |
Ne | 0.3 |
H2 | 0.6 |
N2 | 1.0 |
O2 | 1.7 |
Ar | 2.3 |
Kr | 7.1 |
CO2 | 20.0 |
Xe | 25.6 |
https://en.wikipedia.org/wiki/Nitrogen_narcosis
Avascular necrosis (AVN), also called osteonecrosis or bone infarction, is death of bone tissue due to interruption of the blood supply.[1] Early on, there may be no symptoms.[1] Gradually joint pain may develop which may limit the ability to move.[1] Complications may include collapse of the bone or nearby joint surface.[1]
Risk factors include bone fractures, joint dislocations, alcoholism, and the use of high-dose steroids.[1] The condition may also occur without any clear reason.[1] The most commonly affected bone is the femur.[1] Other relatively common sites include the upper arm bone, knee, shoulder, and ankle.[1]Diagnosis is typically by medical imaging such as X-ray, CT scan, or MRI.[1]Rarely biopsy may be used.[1]
Treatments may include medication, not walking on the affected leg, stretching, and surgery.[1] Most of the time surgery is eventually required and may include core decompression, osteotomy, bone grafts, or joint replacement.[1] About 15,000 cases occur per year in the United States.[4]People 30 to 50 years old are most commonly affected.[3] Males are more commonly affected than females.[4]
https://en.wikipedia.org/wiki/Avascular_necrosis
Decompression sickness (abbreviated DCS; also called divers' disease, the bends, aerobullosis, and caisson disease) is a medical condition caused by dissolved gases emerging from solution as bubbles inside the body tissues during decompression. DCS most commonly occurs during or soon after a decompression ascent from underwater diving, but can also result from other causes of depressurisation, such as emerging from a caisson, decompression from saturation, flying in an unpressurised aircraft at high altitude, and extravehicular activity from spacecraft. DCS and arterial gas embolism are collectively referred to as decompression illness.
Since bubbles can form in or migrate to any part of the body, DCS can produce many symptoms, and its effects may vary from joint pain and rashes to paralysis and death. Individual susceptibility can vary from day to day, and different individuals under the same conditions may be affected differently or not at all. The classification of types of DCS by its symptoms has evolved since its original description over a hundred years ago. The severity of symptoms varies from barely noticeable to rapidly fatal.
Risk of DCS caused by diving can be managed through proper decompression procedures and contracting it is now uncommon. Its potential severity has driven much research to prevent it and divers almost universally use dive tables or dive computers to limit their exposure and to monitor their ascent speed. If DCS is suspected, it is treated by hyperbaric oxygen therapy in a recompression chamber. Diagnosis is confirmed by a positive response to the treatment. If treated early, there is a significantly higher chance of successful recovery.
In 1960, Golding et al. introduced a simpler classification using the term "Type I ('simple')" for symptoms involving only the skin, musculoskeletal system, or lymphatic system, and "Type II ('serious')" for symptoms where other organs (such as the central nervous system) are involved.[1]
The term dysbarism encompasses decompression sickness, arterial gas embolism, and barotrauma, whereas decompression sickness and arterial gas embolism are commonly classified together as decompression illness when a precise diagnosis cannot be made.[6] DCS and arterial gas embolism are treated very similarly because they are both the result of gas bubbles in the body.[5]The U.S. Navy prescribes identical treatment for Type II DCS and arterial gas embolism.[7] Their spectra of symptoms also overlap, although the symptoms from arterial gas embolism are generally more severe because they often arise from an infarction(blockage of blood supply and tissue death).
While bubbles can form anywhere in the body, DCS is most frequently observed in the shoulders, elbows, knees, and ankles.
https://en.wikipedia.org/wiki/Decompression_sickness
Dysbaric osteonecrosis or DON is a form of avascular necrosis where there is death of a portion of the bone that is thought to be caused by nitrogen embolism(blockage of the blood vessels by a bubble of nitrogen coming out of solution) in divers.[1] Although the definitive pathologic process is poorly understood, there are several hypotheses:
- Intra- or extravascular nitrogen in bones, "nitrogen embolism".[citation needed]
- Osmotic gas effects due to intramedullary pressure effects.[citation needed]
- fat embolism[citation needed]
- hemoconcentration and increased coagulability.[citation needed]
No comments:
Post a Comment